Chapter 7
Electromagnetic Compatibility Considerations
7.1 Introduction
Body area communications makes an electromagnetic field source just on or in the human body. Electromagnetic radiation from a body area communication device may give rise to energy absorption in the human body as well as possible interference with medical devices. This implies a potential for possible biological effects as well as malfunction of medical devices that are worn by or implanted in a human body. Both of these electromagnetic compatibility (EMC) issues have to be considered in the design of a body area communication system.
Biological effects caused by exposure to RF fields are related to the electric and magnetic fields inside the human body. The distribution of the internal fields is related to a number of parameters, including the communication frequency, the dielectric properties of tissues, the geometrical shape of the human body, and the antenna structure of the transmitter of body area communications. The interaction of the induced electromagnetic fields with the human body results in the flow of electric current, the polarization of bound charge (formation of electric dipoles), and the reorientation of electric dipoles already present in human tissue. The electrical conductivity governs the flow of electric current, and the permittivity governs the magnitude of polarization effects.
Current knowledge tells us that there are mainly two types of biological effects of electromagnetic fields. One is the stimulation effect to nervous system due to the induced current in tissue. The other is the thermal effect due to energy absorption in tissue. Low frequency electromagnetic fields normally result in negligible energy absorption and no measurable temperature rise in the human body. However, when the frequency exceeds 100 kHz, the stimulation effect of current grows weak because the cell membrane is approximately short-circuited, whereas a significant energy absorption and corresponding temperature rise may occur. In view of this feature, we only need to quantify the energy absorption in body area communications to consider the possible biological effects.
The quantification of energy absorption in the human body is known as dosimetry. The most important quantity of dosimetry at body area communication frequencies is SAR. SAR is the mass averaged rate of energy absorption in the human body
(7.1)
where U is the absorbed energy and m is the mass. In other words SAR is the absorbed power per unit mass in units of watts per kilogram. As described in Chapter 2, it is related to the internal electric field E (root mean square value) by
where is the conductivity of tissue in S/m and
is the mass density in kg/m3.
Since body area communications operate on or in the human body, the safety of the human body has a higher priority than in other wireless communications. This is also one of the major issues to be addressed by IEEE 802.15.6 standard. It has been known that RF energy absorption can result in heating of the human body and an increase in body temperature. If the absorbed energy is greater than the heat released by the human body into the environment, the temperature of the interior of the human body will rise. Tissue damage may emerge primarily because of the body's inability to cope with or dissipate the excessive heat. When the body temperature rises from its normal value, an adverse biological effect may occur. It is therefore essential to limit the transmitting power to as low as possible in order to ensure the safety of humans in body area communications.
On the other hand, electromagnetic interaction from body area communication signals may interfere with body-worn or implanted medical devices to cause a malfunction. A typical implanted medical device is the cardiac pacemaker. The cardiac pacemaker is normally connected to the heart by an electrode to read the ECG signal and to stimulate the heart beat by voltage pulses if necessary. External electromagnetic fields from body area transmitters can couple with the cardiac pacemaker to cause an interference voltage in the internal electronic circuit. If the interference voltage exceeds a threshold in the cardiac pacemaker, the pulse voltage to stimulate the heart beat may be triggered to yield a malfunction. Both electromagnetic interference (EMI) evaluation and the EMC design for medical devices are important in body area communications.
This chapter consists of two main parts which will present the energy absorption in the human body and EMI analysis for a cardiac pacemaker for body area communication signals. For the first issue, we will describe methods to calculate the SAR for both sinusoidal signals and UWB pulse signals. Moreover, we will also discuss the SAR addition effect because multiple transmitters may be used simultaneously in body area communications. For the second issue, we will describe the basic EMI mechanism in the cardiac pacemaker and present a two-step approach to analyze the produced EMI voltage at the cardiac pacemaker. The first step is to calculate the input voltage at the pacemaker circuit using a full-wave electromagnetic field analysis tool by modeling the pacemaker as a receiving antenna, whereas the second step is to analyze the output voltage of the pacemaker circuit to quantitatively evaluate the EMI level. Based on the understanding of EMI mechanism, we will give some guidelines for the EMC design of cardiac pacemakers.
7.2 SAR Analysis
7.2.1 Safety Guidelines
Safety guidelines for RF electromagnetic field exposure are based on the results of critical evaluations and interpretations of the relevant scientific research. From the evaluations, a threshold SAR is established for the most sensitively confirmed biological effect which could be considered harmful to humans. To account for uncertainties in the data and to increase confidence that the guideline is below the levels at which adverse effects could occur, the resulting threshold is usually lowered by a safety factor of 10–50 times below the observed threshold.
The International Commission on Non-Ionizing Radiation Protection (ICNIRP) publishes safety guidelines for RF exposure (ICNIRP, 1998). The physical quantities used to specify the basic restriction are SAR and current density. Protection against adverse biological effects requires that these basic restrictions are not exceeded. According to ICNIIRP, the basic restriction is provided on current density to prevent effects on nervous system functions between 1 Hz and 10 MHz, and on SAR to prevent whole-body thermal stress and excessive localized tissue heating between 10 MHz and 10 GHz. The latter frequency range is of interest for body area communications.
Scientific literature has shown that most confirmed biological effects are associated with significant temperature rise in tissue. Laboratory research with rodents has demonstrated the range of adverse biological effects, from physiological changes to disruption of learned behavioral tasks, resulting from either whole-body or partial-body temperature rises in excess of 1–2 °C. A body temperature rise of 1 °C may be induced by RF exposure for about 30 min at a whole-body average SAR of between 1 W/kg and 4 W/kg. At lower exposure levels, however, there is no convincing evidence for the adverse effects, although sensitive studies can detect adaptive responses such as increased sweating or decreased metabolic rate. In the absence of any convincing evidence for so-called “nonthermal” effects at long-term low-level exposure, modern safety guidelines are based on short-term thermal effects. The threshold for the thermal effects is found to be greater than 4 W/kg even in the most sensitive tissues. A basic restriction for the whole-body average SAR is therefore established at 0.4 W/kg with a safety factor of 10 times for occupational exposure. An additional safety factor of 5 is further introduced for exposure to the general public, giving an average whole-body SAR of 0.08 W/kg.
In contrast to the whole-body average SAR, however, the localized spatial peak SAR is more meaningful in body area communications because the corresponding RF exposure by a transmitter is a highly localized one. This means that the high SAR concentrates in the vicinity of the transmitter, whereas the whole-body SAR is so low as almost negligible. The local heating will be carried to other parts of the human body by blood flow. Hence, the body temperature rise does not only depend on the localized SAR but also on the blood flow. Since the blood flow varies in a complicated manner with body temperature, accurate estimation of temperature rise for a localized exposure is difficult. The ICNIRP also gives a basic restriction for localized SAR as averaged over 10 g of tissue. However, the basis for the threshold of localized SAR is not so clear. Under local exposure conditions, significant thermal damage can occur in sensitive tissues such as the eye and the testis. An SAR from 100 to 140 W/kg at rabbits' eyes was observed to yield a temperature as high as 41–43 °C which caused cataracts. This suggests that a localized SAR of 10 W/kg in eyes will not cause a temperature rise greater than 1 °C. It is thus reasonable to choose 10 W/kg as a basic restriction for localized SAR for occupational exposure. For the general public, moreover, an additional safety factor of 5 yields a localized SAR of 2 W/kg as the basic restriction.
Table 7.1 summarizes the basic restrictions for whole-body SAR and localized SAR between 10 MHz and 10 GHz. All SAR values are averaged over any 6-min period in order to reach a steady state of temperature. For localized SAR, the averaging mass is any 10 g of tissue, and the maximum SAR so obtained is the value used for the estimation with the basic restriction. In addition, for pulse signals of duration T, the equivalent frequency to apply in the basic restrictions is suggested to be calculated as . Moreover, for pulsed exposure in the frequency range of 0.3–10 GHz and for localized exposure to the head, an additional basic restriction is recommended in order to limit or avoid the microwave hearing effect (Lin, 1978). The microwave hearing effect is attributed to a thermo-elastic interaction in the auditory cortex of the brain. The auditory sensation is described as a buzzing, clicking or popping sound, depending on the modulation characteristics of the pulsed field. The basic restriction for pulsed electromagnetic field employs the specific energy absorption (SA) in units of joules per kilogram. The corresponding SA should not exceed 10 mJ/kg for occupational exposure and 2 mJ/kg for the general public, also averaged over 10 g of tissue. In addition, beyond our interested frequency range, between 100 kHz and 10 MHz, which may be also used in HBC, an additional basic restriction for the current density in the head and trunk is required. The corresponding root mean square value of the current density is f/100 mA/m2 where f is the frequency in hertz.
Table 7.1 Basic restrictions for whole-body SAR and localized SAR between 10 MHz and 10 GHz.
Furthermore, body area communications may simultaneously employ multiple frequencies to form a network. In this case, the total SAR is the sum of the SAR at each frequency because it is related to the power.
7.2.2 Analysis and Assessment Methods
It is usually difficult to measure the SAR directly in a living human body, and therefore dosimetry efforts are forced to rely on computer simulation with numerical human body models or experimental simulation with tissue-equivalent phantoms in order to evaluate human safety for body area transceivers.
7.2.2.1 Numerical Techniques
An anatomically based human body model is essential for numerical dosimetry. Such numerical models have been described in Section 3.4 and are especially useful in the FDTD method. For narrow band body area communication signals, the SAR can be evaluated at the carrier frequency. It is straightforward to calculate the SAR at a specific location in the human body for sinusoidal signals because the FDTD method gives the three components of the electric field in each discretized cell. However, the electric fields are given at the edges of each cell in the FDTD method, whereas the tissue types are defined in terms of the cell itself. In order to obtain the SAR in each cell, we need to place all the electric field components in the center of the cell. Figure 7.1 shows the concept for summation of the 12 electric field components, which correctly place all field components in the cell's center to obtain ,
and
, which are the amplitudes of the three electric field components. Then
Figure 7.1 Summation of the field components with the 12-component approach

(7.3)
This approach should be the most reasonable for calculating the SAR in each cell.
In order to assess the safety for the localized exposure by the body area transceivers, we need to calculate the 10-g averaged spatial peak SAR as defined in the basic restrictions of safety guidelines. The whole-body average SAR usually does not need to be considered in body area communications in view of its highly localized exposure and consequent low average of energy absorption in the whole body.
Local SAR assessment sometimes leads to a question of interpretation of the appropriate volume over which the SARs should be averaged in the FDTD method. The specified volume is 10 g of tissue in the shape of a cube or 10 g of contiguous tissue. Since the human body is irregular in shape and is made up of different tissues, finding a precise 10-g cube of tissue around the peak SAR values is often impossible. In addition, due to the difference in mass density of tissues, even cubic volumes of the same size may still yield different mass. Moreover, the FDTD cell size is not always divisible into exactly 10-g cubes.
A possible method to cope with this problem is to use interpolation or extrapolation of the SAR data. For tissue in the body, the spatial peak SAR should be calculated in a cubic volume which contains a mass within ±5% of 10 g. The cubic volume is first centered at each location in the body, and is then expanded in all directions until the desired value for the required mass is reached with no surface boundary of the averaging volume extending beyond the most exterior surface of the body, as shown in Figure 7.2 (IEEE, 2002). For the case of body surface locations, the averaging volume should be constructed with the location centered at one surface of the cube and the other five surfaces of the cube should be expanded evenly in all directions until the required 10 g of tissue in the body is enclosed within this volume.
Figure 7.2 The left cube shows an averaging volume centered on the highlighted cell. The average SAR value is obtained from the enclosed cells and assigned to the highlighted location. The right cube shows an invalid averaging volume(IEEE, 2002). Reproduced with permission from IEEE Std. C95.3-2002 (2002): IEEE recommended practice for measurements and computations of radio frequency electromagnetic fields with respect to human exposure to such fields, 100 kHz–300 GHz
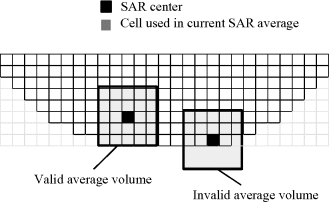
With the local SAR in each cell, a linear interpolation algorithm can be used to derive the 10-g averaged SAR for safety assessment. According to the above-described expanding rule for cubic volumes, a sequence of cubic volumes of increasing size is obtained. The mass-average SAR is then calculated based on the power deposited in two consecutive cubic volumes, from the sequences which have lower and higher mass than the required 10 g, as shown in Figure 7.3. A weighted average between the two cubic volumes is calculated by implementing the following formula (Caputa, Okoniewski, and Stuchly, 1999)
Figure 7.3 Interpolation for obtaining an accurate 10-g averaged SAR. The gray area is the core volume with a mass mc smaller than 10 g. The extra volume with a mass me is between the dotted lines and the gray area (Caputa, Okoniewski, and Stuchly, 1999). Reproduced with permission from Caputa K., Okoniewski M. and Stuchly M.A., “An algorithm for computations of the power deposition in human tissue,” IEEE Antennas and Propagation Magazine, 41, 4, 102–107, 1999. © 1999 IEEE
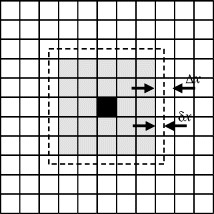
(7.4)
where SAR10g is the 10-g averaged SAR, mg is the required mass of 10 g, Pc is the power deposited in the volume of the lower mass, mc is the mass of that volume, Pe is the power deposited in the extra part of cubes in the next larger volume of the sequence, and me is the mass of that part.
The maximum value of the 10-g averaged SAR obtained in such a way can be used as the required quantity in the safety assessment with the basic restrictions.
On the other hand, for wide band communication signals such as UWB signals, the frequency-dependent FDTD method is usually used in numerical analysis. Since the UWB signals produce pulsed fields, both the SA and the SAR are required for the safety assessment. Here we show two approaches for the SA and SAR calculation.
The first approach is a time-domain approach. Referring to Section 3.1.5, it calculates the current density in the time domain in the frequency-dependent FDTD method by
(7.5)
Then the SA can be obtained from
(7.6)
where T is the pulse duration. This calculation is straightforward in the frequency-dependent FDTD method, and it does not need additional calculation burden.
The second approach is essentially a frequency-domain expression of the first approach. According to Parserval theorem,
(7.7)
where
(7.8)
is the lossy component of the dielectric properties and E(ω) is the Fourier transfer of electric field E(t). We can get E(ω) in the frequency-dependent FDTD method as a running summation at each time step based on the discrete Fourier transfer such as
(7.9)
where Δt is the time step, N is the number of total time steps, and . This approach requires additional calculation burden.
Although the corresponding numerical algorithms are different, the two approaches are mathematically equivalent. Let us consider a simple example for comparison of the two approaches. A disk dipole antenna is placed in the front of a homogenous cube, and is excited by a UWB pulse signal. The cube has a length of 200 mm and the dielectric properties of muscle. Figure 7.4 shows the SA profile, taken from the front to the back of the muscle cube, calculated by using the two different approaches. As can be seen, the two approaches give the same SA values. In view of the calculation efficiency, the first approach in the time domain is more appropriate for the SA calculation in the frequency-dependent FDTD method. As for the SAR, it can be simply obtained from the ratio of the SA to the pulse duration T, that is,
Figure 7.4 Comparison of the calculated SA between the time-domain approach and the frequency-domain approach. The two approaches give the same result so that the two curves completely overlap

7.2.2.2 Measurement Techniques
Experimental dosimetry is focused mainly on homogeneous, tissue-equivalent phantoms, because of the difficulty in developing a heterogeneous human body model. Phantoms generally simulate a single tissue such as muscle, or a mixed-tissue such as the body, by having the same permittivity and conductivity as the actual tissue. Phantoms can be mainly classified into three types depending on the phantom form: liquid, solid, or gel.
A liquid phantom permits scanning of the electric fields inside it using an electric field probe, which can provide high-precision SAR measurement. The main materials in most liquid phantoms are deionized water, sugar, and sodium chloride. Also other materials such as hydroxyethyl cellulose are often used to adjust the permittivity and conductivity. The permittivity is adjusted mainly by varying the percentage of sugar, and the conductivity is adjusted mainly by altering the percentage of sodium chloride. Because it is based on water, a liquid phantom is generally good at simulating a high water content tissue. A mixture of a physiological salt solution and ethylene glycol enables the liquid phantom to also simulate a low water content tissue. The drawback of liquid phantoms is the evaporation of water, which changes the permittivity and conductivity. This, however, can be managed by the appropriate replenishment of water. A possible composition at 400 MHz for muscle tissue is shown in Table 7.2 (Hartsgrove, Kraszewski, and Surowiec, 1987).
Table 7.2 Composition of liquid phantom (wt%) for muscle at 400 MHz.
In liquid phantoms, the SAR assessment is usually conducted by measuring the electric field. The SAR is calculated from the measured electric fields with Equation 7.2. The main requirements for an electric field probe in SAR measurement are: (1) high sensitivity and linear response over a broad frequency range; (2) high spatial resolution; (3) isotropy in different media; (4) low interaction with the measured field; and (5) small size. Most existing electric field probes are based on Schottky diode detectors. The measured signal at the probe output is a voltage proportional to the electric field E or the squared electric field E2.
Because the SAR assessment requires all three components of the electric field, most electric field probes consist of three small dipoles, with detector diodes at their center gaps. A triangular-beam structure, as shown in Figure 7.5 (Schmid, Egger, and Kuster, 1996), is a typical design because of its small size and the possibility of placing the detector in the center of the probe. The probe consists of three sensors. Each sensor consists of (1) a short dipole antenna, (2) a diode detector at the dipole feed-gap, (3) a dielectric mechanical support, and (4) a highly resistive feed-line to extract the signal detected by the diode to the measuring unit. The probe's tip length and tip diameter are on the order of 2 cm and 1 cm, respectively. This permits a high spatial resolution for scanning electric fields within a liquid phantom. Such probes may typically have a frequency range from 10 MHz to 10 GHz, a dynamic range of 5 μW/g to 100 mW/g, and an orthogonal directivity pattern for the three sensors.
Figure 7.5 A typical electric field probe with a triangular beam structure. Schmid, Egger, and Kuster, 1996

Probe calibration for SAR measurement in liquid phantoms will give either an SAR or an electric field conversion factor. Because SAR is proportional to liquid conductivity, a direct calibration in terms of SAR is valid only for liquids with exactly the same conductivity. The electric field sensitivity depends more on both the liquid permittivity and the liquid conductivity and therefore is less sensitive to the conductivity alone. Calibration in terms of electric field, rather than SAR, should have a broader range of validity. Possible calibration methods include both waveguide calibration and thermal calibration. In a waveguide calibration, the measured fields are compared with analytical solutions. In the thermal calibration, the SAR values are compared with the temperature rise. The former is preferable, because it gives higher precision.
In addition to liquid phantoms, solid and gel phantoms are also useful in SAR assessment. One representative solid phantom is the TX-151 phantom (Ito et al., 1998). A TX-151 phantom is applicable for simulation of a high water content tissue in the microwave band. The permittivity is adjusted mainly by the amount of polyethylene powder mixed in agar, and the conductivity is adjusted mainly by the amount of sodium chloride. TX-151 acts as an adhesive in the agar liquid; thus, it forms a solid phantom. The materials required are easily obtained, and it is easy to make TX-151 phantoms of differing shapes. If the phantom is wrapped with vinyl film, it is possible to maintain constant permittivity and conductivity for 1 month at room temperature. Glycerin-based phantoms can provide a longer life span than TX-151. Their life span can be extended to 6 months by wrapping the phantom with vinyl film because glycerin acts to maintain humidity. The permittivity in a glycerin-based phantom is adjusted mainly with deionized water, and the conductivity is adjusted with sodium chloride. Polyethylene powder is used in fine adjustment of the permittivity and conductivity. Compared with the TX-151 phantom, the drawback of a glyceric phantom is the narrower frequency range for a single composition. At different frequencies the percentages of the various materials have to be changed.
Gel phantoms are typically manufactured from a physiological salt solution and polyethylene powder mixed with TX-150 or agar. They are used to simulate a high water content tissue. With a gel phantom, it is difficult to have a fixed shape, unless a container is used.
Scanning the electric fields in a solid or gel phantom for SAR assessment is impractical. They are more appropriate for measuring the temperature rise. Under the assumption of linear energy deposition over a fixed period of time, the SAR can be determined from
where Cp is the specific heat of the phantom, is the temperature rise caused by the exposure, and
is a very short exposure time.
There are two methods for the measurement of temperature. One method is to use a flour-optic temperature probe. These probes utilize temperature-dependent fluorescent decay or interferometric micro shifts of cavity resonators. One can insert a flour-optic temperature probe into a phantom and measure the temperature over a period of time; this allows a history of temperature rise over time to be recorded. The second method is to use an infrared image camera. The solid or gel phantom, before exposure, is first set at the ambient temperature, and the corresponding infrared image is recorded. Immediately after the electromagnetic exposure, another infrared image is taken. Then the temperature rise at the phantom surface is obtained from the temperature difference, and the SAR is determined from Equation 7.11. However, the exposure time for thermal measurement has to be short enough to prevent heat transfer from phantom to air. In order to keep a linear temperature rise over a short period of time requires use of a high-output power amplifier. More than 10 W is usually required to produce an efficient temperature rise of 1–2 °C within 1 min. The thermal method is therefore a weak approach when measuring low SAR values, which is actually a typical situation in body area communications. The precision of thermal measurement is also poorer than the electric field probe approach.
In view of these observations, a numerical analysis approach with realistic-shaped human body models or a measurement approach with electric field probe scans in a liquid phantom is the appropriate choice for SAR assessment in body area communications.
7.2.3 Transmitting Power versus SAR
7.2.3.1 On-Body UWB
UWB communication is a candidate for on-body applications. A typical UWB antenna may be an elliptic disk dipole with a major axis radius of 12 mm and a minor axis radius of 10 mm. As shown in Figure 7.6, such a structure yields a VSWR of nearly 2.0 between 3.1 GHz and 10.6 GHz. In order to make a quantitative analysis for the SA and SAR in a human body for an actual UWB transmitter, we employ this antenna in an on-body communication scenario.
Figure 7.6 An elliptic disk dipole antenna and its VSWR
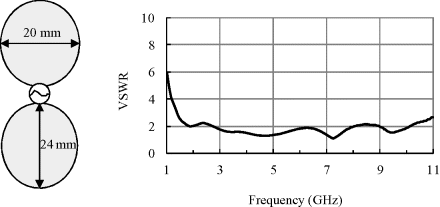
The antenna is assumed to have a spacing of 2 mm from the human body surface. The UWB pulse to be transmitted is considered as a Gaussian derivative because it can be generated in the easiest way by a real pulse generator and be radiated in an efficient way. Figure 7.7 shows a fifth-derivative Gaussian pulse waveform with a width of nearly 500 ps, and its EIRP. The EIRP is the amount of power that a theoretical isotropic antenna (which evenly distributes power in all directions) emits to induce the peak power density observed in the direction of maximum antenna gain. It is derived as follows
Figure 7.7 (a) Fifth-derivative Gaussian pulse waveform and (b) its EIRP versus frequency
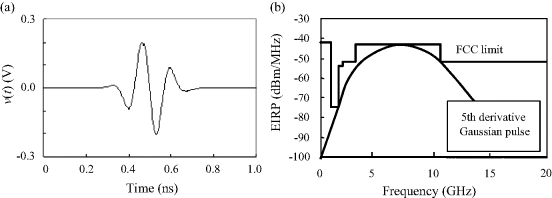
(7.12)
where Pa is the power supplied to the UWB antenna and Ga is the maximum absolute gain of the antenna. The determination of Pa depends on the UWB modulation scheme. For IR-UWB, we can first calculate the antenna power density
(7.13)
where V(f) is the Fourier transform of the UWB pulse voltage in units of V/Hz, Zin (f) is the antenna input impedance and Tp is the pulse duration. Then we have the EIRP as
(7.14)
where the number 90 is introduced by the units of mW and MHz. It can be found from Figure 7.7 that the maximum power density of the employed fifth-derivative Gaussian pulse is limited to −43.1 dBm/MHz between 3.1 GHz and 10.6 GHz, that is, it meets with the FCC indoor emission spectrum mask. Careful adjustment of pulse shape factors for other orders of Gaussian derivatives is also possible to produce a power density which approximately meets with the FCC UWB emission limit. However, the fifth-derivative Gaussian pulse is a superior choice because it closely approximates the FCC emission mask so that more permissible power can be radiated. This is desirable in view of the excitation source requirement in the SA/SAR analysis.
In view of typical on-body communication scenarios, we consider that the UWB antenna is placed at the chest, ear, eye and waist, respectively, parallel to the body surface. Moreover, since in actual on-body applications many transmitters may communicate at the same time, it is necessary to also consider a multiple exposure scenario in order to investigate the possible addition of energy absorption. As a multiple exposure scenario, two UWB antennas are simultaneously placed at the chest and waist, or four UWB antennas are simultaneously placed at the above four locations. Figure 7.8 shows the SA distributions on the body surface for the UWB pulse exposure under the FCC emission limits (Wang and Wang, 2009). The 0 dB corresponds to 10 pJ/kg. As can be seen, the SA concentrates on a very small area on the body surface near the UWB antenna location, and the attenuation is more than 30 dB at a location 10 cm away from the UWB antenna.
Figure 7.8 SA distributions under the FCC UWB limit (approximately 0.5 mW transmitting power) for various antenna locations. The 0 dB corresponds to 10 pJ/kg (Wang and Wang, 2009). Reproduced with permission from Wang Q. and Wang J., “SA and SAR analysis for wearable UWB body area applications,” IEICE Transactions on Communications, E92-B, 2, 425–430, 2009
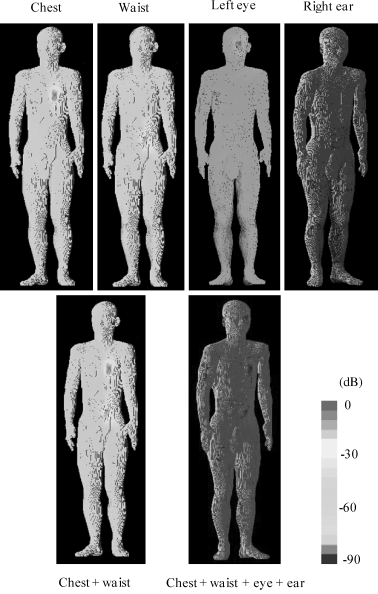
For an IR-UWB pulse signal, the SAR can be simply obtained from the ratio of SA to the pulse duration T (Equation 7.10). It is reasonable because the transmitted signal is in a form of successive pulses in the IR-UWB scheme. Table 7.3 summarizes the 10-g averaged spatial peak SA and SAR in all of the considered transmitter locations under the FCC emission limits. It should be noted that the full UWB band permits a maximum transmitting power of 0.556 mW as described in Chapter 1. Since the actual UWB pulse as shown in Figure 7.7 does not completely fit the shape of the FCC spectrum emission limit, the transmitting power is somewhat smaller than 0.556 mW, typically 3 dB smaller than the maximum power. Here we approximate it as 0.3 mW. As can be seen, under this transmitting power, the 10-g averaged SA is on the order of pJ/kg which is much smaller than the ICNIRP safety guideline of 2 mJ/kg. Moreover, the 10-g averaged SAR is on the order of mW/kg which is smaller than 1/2000th of the ICNIRP safety guideline of 2 W/kg. Besides, the energy absorbed by the whole body is found to be 0.01 pJ which is about a quarter of the energy radiated from the antenna which is around 0.04 pJ.
Table 7.3 10-g averaged peak SA and SAR under FCC UWB pulse emission limit (approximately 0.3 mW transmitting power)
Antenna location | 10-g peak SA (pJ/kg) | 10-g peak SAR (mW/kg) |
Chest | 0.473 | 0.946 |
Ear | 0.037 | 0.074 |
Eye | 0.268 | 0.536 |
Waist | 0.232 | 0.464 |
Chest + waist | 0.474 | 0.948 |
Chest + ear + eye + waist | 0.476 | 0.952 |
Moreover, compared with the SAR for single exposure, almost no obvious increase is observed in the SA and SAR for the multiple exposures from Table 7.3. This can also be observed from the SA distributions in Figure 7.8. In order to further verify this conclusion, let us consider the time waveform of electric field at the chest under multiple exposures. Since the separation distance between two on-body transmitters is about or larger than 20 cm, the transmission time is at least 0.7 ns. This means that if the effect of signals from other transmitters is not negligible at the chest, there should be some significant components after 0.7 ns in the observed electric field waveform. This does not depend on whether the transmitters are working simultaneously or not. From Figure 7.9, however, no significant component is observed over there. This means that as long as the separation between two UWB devices is larger than 20 cm, which is usually satisfied in most cases of on-body communications, the additional effect of signals from other transmitters can be ignored because of the rapid surface attenuation of UWB signals.
Figure 7.9 Electric field waveform at the chest under multiple exposures

In addition, the SA and SAR vary with the distance between the antenna and the human body. Table 7.4 shows the 10-g averaged peak SA and SAR corresponding to distances of 2 mm, 1 cm and 2 cm when the UWB antenna is placed on the chest. It can be seen that the 10-g averaged peak SA and SAR values will decrease by 11 and 16 dB, respectively, when the distances are 1 and 2 cm compared with the initial distance of 2 mm. In practice, the antenna is placed as close as possible to the body surface. The SA and SAR at 2-mm spacing should represent an almost worst case. The further the distance becomes, the larger the safety margin will be.
Table 7.4 10-g averaged peak SA and SAR with different antenna distance from the body.
Antenna spacing from body | 10-g peak SA (pJ/kg) | 10-g peak SAR (mW/kg) |
2 mm | 0.473 | 0.946 |
1 cm | 0.034 | 0.068 |
2 cm | 0.013 | 0.026 |
7.2.3.2 In-Body MICS Band
The MICS band attracts much attention especially in in-body communication such as the capsule endoscope and cardiac pacemaker. Since the implant transmitters are mainly used for medical treatment, the permissible SAR level may be higher than that in daily situations. However, there are no established restrictions for SAR in medical treatment. The safety limit of 2 W/kg for the general public or 10 W/kg for occupational exposure, as averaged over any 10 g of tissue, may be used in in-body communication.
The approach for in-body SAR evaluation may be summarized as follows:
In order to have a quantitative understanding of the SAR at the in-body MICS band, let us consider a capsule endoscope scenario. We assume the implant antenna to be a 2-cm-long dipole. Since the induced SAR will be different for different antenna types, the following result is just an example to demonstrate this analytical approach. In addition, it should be noted that the SAR is defined within 6 min of reaching a thermal equilibrium. A capsule endoscope may stay in one location in the digestive organs more or less than 6 min. In either case, the calculated SAR can be considered as a worst case estimation.
As described in Section 4.2.4, the dipole is moved along the digestive organs, from the esophagus and stomach to the small intestines and large intestines, with a spacing of several centimeters and three directivities of x, y and z, respectively. With the transmitting power as the input to the antenna, the 10-g averaged spatial peak SAR is calculated using the FDTD method in conjunction with the anatomical human body model. Figure 7.10 shows an example of SAR distribution when the antenna is located in the stomach. It can be seen that the higher SAR area is limited in a small region. This is because of the small size of antenna which yields a high field concentration.
Figure 7.10 Normalized SAR distribution when the antenna is located in the stomach in the MICS band
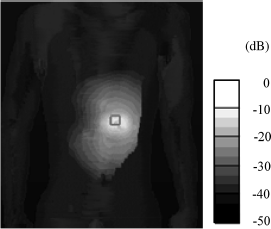
Based on a link budget analysis as described in Section 6.4.2, at each receiver location in Figure 4.11, the required transmitting power is shown in Table 7.5 for all transmitting dipole locations and directivities in the digestive organs to ensure a BER of 10−3 at a data rate of 1 Mbps with BPSK modulation. The required mean transmitting power denotes the power which yields an average BER of 10−3, while the required maximum transmitting power denotes the power which yields the BER always smaller than or equal to 10−3. From Table 7.5, the required mean transmitting power at BER = 10−3 is almost smaller than 20 mW. Although such a transmitting power is larger than that in Figure 6.35 because all the capsule locations are considered, it can never induce a 10-g averaged SAR exceeding 2 W/kg. In this sense the safety guideline is always satisfied. However, if we require a higher communication quality to ensure the BER is always smaller than 10−3 in any capsule location, the SAR will be determined by the maximum transmitting power in Table 7.5.
Table 7.5 Required maximum and mean transmitting power at BER = 10−3 for all capsule locations.
Maximum transmitting power (mW) | Mean transmitting power (mW) | |
Rx1 | 120.71 | 20.85 |
Rx2 | 20.83 | 2.96 |
Rx3 | 52.04 | 5.04 |
Rx4 | 115.10 | 18.25 |
Rx5 | 105.12 | 7.70 |
Moreover, when the receiving antenna is located in the front of the human body, the path loss is relatively small so that the required transmitting power is also small. In order to have a statistical observation for the SAR characteristics, we show the probabilities of the 10-g averaged spatial peak SAR in Figure 7.11 for all transmitting antenna locations and directivities (90 data in total) in the digestive organs. The vertical axis in the figure denotes the percentage of SAR values on the horizontal axis. The SAR values are calculated at the maximum transmitting power for Rx1 and Rx2, respectively. As can be seen, if the receiving antenna is set at the Rx2 location, a transmitting power of 20.83 mW is required to ensure a BER not exceeding 10−3. Such a transmitting power yields a local peak SAR ranging from 0.45 to 1.8 W/kg, which does not exceed the safety guideline of 2 W/kg. On the other hand, if the receiving antenna is set at the Rx1 location, a transmitting power of 120.71 mW is required to ensure a BER not exceeding 10−3. In this case the local peak SAR may always exceed 2 W/kg at any transmitter locations.
Figure 7.11 Probabilities of 10-g averaged peak SAR in digestive organs for receiver locations (a) Rx1 and (b) Rx2 to have a BER always below 10−3
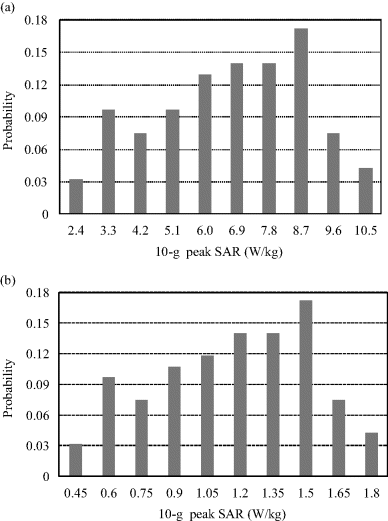
However, in view of the fact that the capsule endoscope is a medical treatment, it should be acceptable to use 10 W/kg as the safety guideline. So the induced local peak SAR satisfies the safety guideline for occupational exposure. Moreover, the results in Figure 7.11 also suggest that the SAR is dependent on the receiver locations. If we can optimally choose a receiver location, for example Rx2, both a BER smaller than 10−3 and a local SAR smaller than 2 W/kg are obtainable.
Another useful expression for SAR statistics is the cumulative distribution function or CDF. Figure 7.12 shows the CDFs of the 10-g averaged peak SAR when the transmitting antenna moves along the digestive organs to have 30 locations and three different directivities. The SAR values are calculated at the maximum transmitting power for receiver locations Rx1, Rx2 and Rx3, respectively. The plots obviously show that the 10-g peak SAR will satisfy the safety guideline of 2 W/kg at Rx2, and 10 W/kg at Rx3. However, if the receiving antenna is set at Rx1, a transmitting power of 120.71 mW is required so that even the safety guideline of 10 W/kg cannot be satisfied in some transmitter locations.
Figure 7.12 Cumulative distribution functions of 10-g averaged peak SAR in digestive organs for securing a BER always below 10−3

An effective technique to improve the BER performance is known as spatial diversity reception. Such a technique can also be used to reduce the SAR. Here we consider a two-branch EGC diversity. As described in Chapter 6 in detail, we first obtain the Eb/N0 versus BER performance for the diversity reception via a theoretical analysis or computer simulation, then we derive the required transmitting power to secure a BER of 10−3 based on the established path loss channel model. The required mean transmitting power and maximum transmitting power are shown in Table 7.6. Compared with the results for the single receiver case, we can see that applying spatial diversity reception to the receivers can significantly reduce both the required mean transmitting power and the required maximum transmitting powers. Furthermore, similar to the single receiver case, the required mean transmitting power is also less than 10 mW at all selections of the receiving antennas, that is, the 10-g averaged SAR will never exceed 2 W/kg. If we can choose a good combination of receiving antenna positions, for example Rx2 and Rx3, the achievable required maximum transmitting power is only 1.54 mW, which is only about 7% of the required maximum transmitting power in the case of a single receiver.
Table 7.6 Required mean and maximum transmitting power at BER = 10−3 for diversity reception.
Figure 7.13 shows the CDFs of the 10-g averaged peak SAR in the case with the spatial diversity reception at the required maximum transmitting power for the combination of Rx2 and Rx3 and the combination of Rx1 and Rx5. From this figure, in addition to the required transmitting power, the SAR is also remarkably reduced compared with that for the single branch case. Even if the two receiving antennas are set to Rx1 and Rx5 (the worst selection), a transmitting power of only 5.19 mW is required to ensure a BER not exceeding 10−3. Such a transmitting power yields a 10-g averaged peak SAR below 0.5 W/kg. This result means that the maximum transmitting power can never yield a 10-g averaged SAR larger than 2 W/kg with a spatial diversity reception. Moreover, if we can optimally select the diversity branches, for example the selection of Rx2 and Rx3, the 10-g averaged peak SAR will have a margin more than 10 dB with respect to the safety guideline of 2 W/kg.
Figure 7.13 Cumulative distribution functions of 10-g averaged peak SAR in digestive organs for securing a BER always below 10−3 with spatial diversity reception
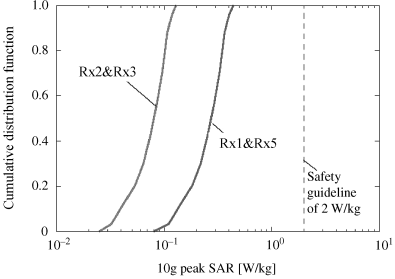
Table 7.7 gives the permissible transmitting power for securing the local SAR not exceeding 2 W/kg or 10 W/kg, which can be used as a threshold transmitting power in safety evaluation for the capsule endoscope transceiver with dipole antenna. Then, if an implant transceiver needs to be evaluated, we can insert it into a liquid phantom of the human body and measure its transmitting power. By comparing the measured transmitting power with the threshold transmitting power, it is possible to evaluate the safety of the transceiver for capsule endoscope application.
Table 7.7 Threshold transmitting power at a local SAR of 2 or 10 W/kg.
Local peak SAR (W/kg) | Transmitting power (mW) |
2 | 24.36 |
10 | 121.80 |
Of course for different transmitter antennas the in-body SAR values may vary. However, the basic approach of SAR evaluation is the same as above. It should be noted that the improvement of receiver performance, for example, to adopt a high-gain receiving antenna or to adopt a spatial diversity reception technique, can effectively reduce the required transmitting power inside the body. This will contribute to the reduction of the peak SAR. As long as the transmitting power is below 20 mW, the 10-g averaged peak SAR will never exceed 2 W/kg.
7.2.3.3 HBC Band
HBC is considered between 10 MHz and 50 MHz in IEEE 802.15.6 standard. A typical HBC transmitter employs an electrode structure on the surface of the human body to transmit signals. In view of a 60 dB average path loss in the HBC band, A 1 V transmitting voltage is required to produce at least 1 mV receiving voltage at the receiver for demodulation. We therefore evaluate the SAR when the electrode is excited with a 1 or 10 V voltage source at 30 MHz.
Consider the human body model in Figure 4.19. The electrode is assumed to be on the left chest or back pocket location. The SAR is then calculated using the FDTD method in conjunction with a homogeneous human body model. The homogeneous human body model is assumed to have a conductivity and permittivity two-thirds that of muscle. This is because a human body has an approximated average composition of two-thirds muscle-like tissue. Figure 7.14 shows the SAR distribution on the human body surface with the transmitting electrode on the chest. The energy absorption is also quite concentrated due to the small size of the electrode. Table 7.8 summarizes a calculation example of the 10-g averaged spatial peak SAR for 1 V as well as 10 V transmitting voltage. Compared with that in the back pocket case, the higher 10-g SAR level is found in the chest due to its flat shape. In total, the 10-g averaged spatial peak SAR is on the order of μW/kg at the usual 1 V transmitting voltage, with a safety factor of more than 60 dB compared with the safety limit of 2 W/kg. The result suggests that it is not difficult to satisfy the SAR guidelines in human body communications.
Table 7.8 10-g averaged peak SAR (μW/kg) for 1 or 10 V transmitting voltage in the HBC band.
1 V | 10 V | |
Chest | 0.99 | 99 |
Back pocket | 0.10 | 10 |
Figure 7.14 SAR distribution on the human body surface in the HBC band
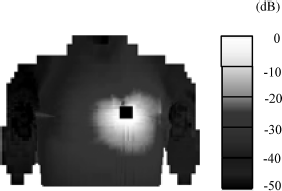
7.3 Electromagnetic Interference Analysis for the Cardiac Pacemaker
7.3.1 Cardiac Pacemaker Model and Interference Mechanism
In addition to the electromagnetic energy absorption issue due to body area communication signals, the electromagnetic interaction of body area communication signals with the human body implies a potential for EMI with implanted medical devices such as a cardiac pacemaker. The cardiac pacemaker consists of a shielded housing with electronic circuits inside and an electrode. It is connected to the heart by the electrode to read the ECG signal and to simulate the heart beat by voltage pulses if necessary. External electromagnetic fields can couple into the pacemaker to cause an interference voltage at the input of the internal sensing circuit. The interference voltage produced at the input of the sensing circuit of pacemaker will be amplified and low-pass filtered. If the output voltage of the amplifier and low-pass filter exceeds a threshold, the pulse voltage to simulate the heart beat may be triggered and a malfunction of the cardiac pacemaker may occur. Although the body area communication signal is generally at higher frequencies (greater than MHz) compared with the working frequency (in the order of kHz) of the pacemaker, the nonlinearity of the internal sensing circuit may produce a demodulation effect so that the RF signals fall into the working frequency band of the pacemaker.
In this section, we will introduce a detailed two-step approach to analyze the EMI voltage induced at the cardiac pacemaker. In the first step, the input voltage of the pacemaker circuit is calculated using the FDTD method by considering the pacemaker as a receiving antenna. In the second step, a Volterra series is employed to analyze the output voltage of the nonlinear amplifier and low-pass filter circuit in the pacemaker for evaluating the EMI effect.
The pacemaker may act as a receiving antenna with respect to the external electromagnetic fields (Wang, Fujiwara, and Nojima, 2000). It is reasonable to apply this behavior to any body area communications frequency (Wang et al., 2009). Figure 7.15 shows a basic configuration for an implanted cardiac pacemaker. The cardiac pacemaker consists of a shielded housing with electronic circuits inside and one (unipolar) or two (bipolar) electrodes as well as the lead wires. Without losing generality, we here consider a unipolar electrode case. By considering the internal impedance seen from the connector to the internal circuit as a load, and the metal portions consisting of the pacemaker housing and the lead wire of the electrode as two elements of a receiving antenna, the resultant equivalent circuit for the pacemaker can be shown as in Figure 7.16. Here, is the radiation impedance of the pacemaker,
is the open voltage induced between the pacemaker housing and the lead wire due to the electromagnetic fields from the external communication devices,
is the internal impedance of the pacemaker seen from the connector, and
is the voltage produced through the connector onto the internal sensing circuit, which is referred to here as the input interference voltage to the internal sensing circuit of the pacemaker. Then the input interference voltage
of the sensing circuit can be obtained as
Figure 7.15 Basic configuration of a cardiac pacemaker

Figure 7.16 Equivalent circuit for EMI predication
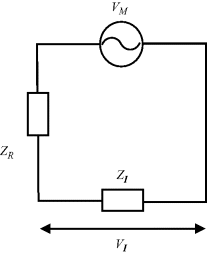
Figure 7.17 shows the block diagram of the analog sensing circuit of the pacemaker (Tarusawa et al., 2005; Schenke, Fichte, and Dickmann, 2007). The input voltage is amplified and low-pass filtered. The resulting output voltage
is compared with a sensing threshold
. When the voltage exceeds the sensing threshold, it will switch on the pulse output, and then create a malfunction in the pacemaker.
Figure 7.17 Block diagram of the internal pacemaker circuit

Whether a body area communication signal can pass through the low-pass filter depends on its characteristic parameters such as the signal magnitude, frequency component, modulation scheme as well as the parameters of the sensing circuit. Figure 7.18 illustrates the basic concept of EMI caused by a RF communication signal. An ECG signal usually ranges from 1 Hz to 1 kHz. The electric circuit of a pacemaker is thus principally designed to work in this low frequency band so that it is difficult for a RF interference signal to appear in the input to the comparator. However, if the RF interference signal is sufficiently strong, the pacemaker circuit may behave nonlinearly. The nonlinearity comes from either the feed through filter or the amplifier (Barbaro et al., 2003). After passing through the feed through filter, the RF signal is impressed to the amplifier for ECG sensing. Since the interference signal frequency is usually beyond the working range of the amplifier, the interference signal will be weakened but the amplifier's nonlinearity may produce a new DC offset and low frequency components as shown in Figure 7.18(b). The DC and low frequency components can pass through the low-pass filter and become the input of the comparator as shown in Figure 7.18(c). If the comparator cannot efficiently reject this interference signal VO, the pacemaker may malfunction when VO is larger than the threshold voltage Vt.
Figure 7.18 Basic concept of EMI caused by a body area communication signal
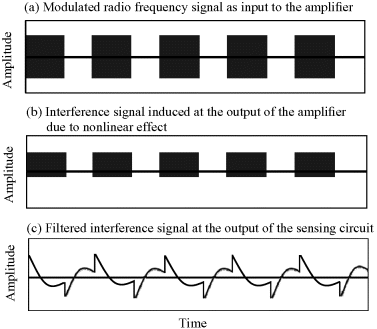
The nonlinearity of an analog sensing circuit can be analyzed using the Volterra series method which is a classical and powerful tool to describe a weakly nonlinear circuit. Based on the nonlinear circuit model, the output interference voltage VO can be derived to predict the potential interference to the pacemaker.
This two-step approach for analyzing pacemaker EMI can be outlined as follows:
Now let us introduce the electromagnetic field approach and electric circuit approach in Sections 7.3.2 and 7.3.3, respectively.
7.3.2 Electromagnetic Field Approach
The open voltage at the connector can be obtained by modeling the pacemaker as a receiving antenna with an open load at the connector using the FDTD method. The receiving antenna has a dipole structure in which the two radiating elements are the metal housing and the metal lead line of electrode. Using the FDTD method, the open voltage
between the metal housing and the lead wire, that is, at the connector, can be obtained when the transmitter electrode is excited, and then the interference voltage at the input of the internal pacemaker sensing circuit can be estimated.
The detailed steps for the electromagnetic field approach are as follows:









(7.17)
7.3.3 Electric Circuit Approach
A pacemaker sensing circuit is composed of an amplifier and a low-pass filter. A typical circuit can be considered as an operational amplifier (opamp) combined with external elements. An opamp is generally a high-gain electronic voltage amplifier with a differential input and a single-ended output. Figure 7.19 shows a representative negative feedback opamp configuration with differential inputs. As described above, although the body area communication signal is generally at higher frequencies compared with the heart-beat-stimulating pulse signal, the opamp's nonlinearity may convert the high frequency signal to lower frequencies and enable it to pass through the low-pass filter. Measured results in Schenke, Fichte, and Dickmann (2007) also show that the ideal linear opamp model is no longer applicable at higher frequency due to the demodulation property of the opamp. A nonlinear model is therefore necessary for an opamp with low-pass filter character in order to accurately predict the interference output. Although the feed through filter before the opamp may also yield a nonlinear result, we here focus the nonlinear analysis on the opamp.
Figure 7.19 General negative feedback opamp configuration with differential inputs

The Volterra series is known as a powerful and accurate tool for weakly nonlinear time-invariant analog circuit analysis (Schetzen, 1980). It is nowadays extensively used to calculate small, but nevertheless troublesome, distortion terms in transistor amplifiers and systems. Compared with the Taylor series, it is characterized by the ability to capture “memory” effect which implies the output of the nonlinear system depends on the input to the system at all other times. This effect is beneficial to model the nonlinear behavior in “memory” effect devices such as capacitors and inductors. In mathematics, the Volterra series represents a functional expansion of a dynamic, nonlinear, time-invariant function and is a series of infinite sum of multidimensional convolution integrals.
It is known that a linear time-invariant system with memory can be described by the convolution representation
(7.18)
where is the input signal,
is the output signal and h(t) is the impulse response of the system.
According to the Volterra series method, the output signal of a nonlinear time-invariant system with memory can be expressed in the form (Schetzen, 1980)
(7.19)
where
(7.20)
is the Fourier transform of the system input and
with
(
is the potential highest order of the system) are the ith order frequency-domain Volterra kernels. If the system is considered as a second-order system
, the
item will contain
,
,
,
,
and
. The Volterra series method depicts that any nonlinear system, in principle, can be modeled through multi-dimensional convolution. However, in practice, the kernels are usually only considered up to order 3 or rarely 5 due to complex kernels. As a result of this limitation, this method is only used for weakly nonlinear systems.
For a weakly nonlinear negative feedback opamp driven by RF interference, the output voltage expression for two arbitrary input signals has been derived using the second-order Volterra series (Fiori and Crovetti, 2003). Based on the derived Volterra kernels in Fiori and Crovetti (2003) and with input voltage in the pacemaker case, we can get the opamp output voltage as follows
where and
are the frequency domain Volterra kernels, which depend on the opamp parameters and the external components. With
in the pacemaker case, the negative feedback opamp in Figure 7.19 can be simplified as in Figure 7.20. Therefore, Equation 7.21 is a general second-order nonlinear opamp output voltage expression for an internal pacemaker sensing circuit. In terms of the weak nonlinearity of the Volterra series, we assume the opamp of the pacemaker internal circuit to be a second-order nonlinear system.
Figure 7.20 An opamp model for an internal pacemaker sensing circuit
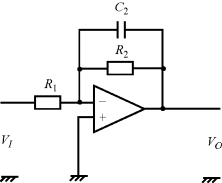
Next we give the frequency domain Volterra kernels and
in Equation 7.21.
(7.22)
(7.23)
(7.24)



(7.25)
(7.26)
(7.27)
(7.28)
(7.29)
(7.30)
(7.32)
(7.33)




(7.34)
(7.35)
(7.36)
(7.37)
(7.38)
(7.39)
(7.40)
(7.41)
The derivation of the Volterra kernels used for the second-order nonlinear opamp has been presented in detail. In short, the Volterra kernels depend on the electrical parameters of the opamp components. If the input interference to the internal pacemaker sensing circuit is also known, we can then get the output interference signal based on the Volterra nonlinear model in Equation 7.21.
Based on the above two approaches, the prediction of EMI voltage at the analog sensing circuit output of a pacemaker can be now summarized as follows:








7.3.4 Transmitting Signal Strength versus Interference Voltage
With the above-described method, in this section, we will focus on two types of on-body communication signals as the external interference sources to analyze the potential interference voltage at the implanted pacemaker output. From the perspective of the interference signal type, the carrier signal and pulse signal are two representative transmission signals. For the carrier signal, we will give an example in the HBC band, while for the pulse signal, the UWB band is naturally used. They actually correspond to narrow band and wide band communication signals, respectively.
7.3.4.1 Narrow Band HBC Signal
It has been described in Chapters 2 and 4 that the propagation in the HBC band is mainly based on an electrostatic coupling or approximate surface wave. The dielectric properties of human tissue determine that such a transmission should be expected in a frequency below dozens of MHz. On the other hand, however, the electromagnetic interaction of on-body communication signals with the human body is significant in this frequency range because of the easier penetration of electromagnetic fields into the human body. This implies a potential EMI with an implanted cardiac pacemaker.
For a narrow band on-body HBC communication signal at carrier frequency , the input interference voltage can be approximated in a delta-function form
. Based on Equation 7.21, the output voltage at the sensing circuit can be written as
where is the amplitude of the input narrow band signal, and
and
are the frequency-domain Volterra kernels, which depend on the opamp parameters and the external components. Since the term at
(in MHz) is beyond the opamp circuit bandwidth (in kHz), only the second term in Equation 7.43 is effective after the low-pass filter. This indicates that the EMI effect is actually caused by an offset voltage in the output. The interference output voltage produced by the narrow band HBC signal can then be rewritten as
(7.44)
Based on the derivation of H22 (fc, −fc) from Equations 7.31 to (7.42), the following is obtained
(7.45)
Then the interference output voltage VO is
According to Schenke, Fichte, and Dickmann (2007), the low-pass filter of the pacemaker circuit has a cut-off frequency of 1 kHz which means . Assuming a 10 dB gain of the negative feedback opamp circuit, the component parameters for the circuit in Figure 7.20 can be determined as
,
and
. Furthermore, with typical values of
and
, we can obtain the output voltage V0 for a known interference input voltage at the pacemaker sensing circuit.
The measured values given in Schenke, Fichte, and Dickmann (2007) show a critical input voltage of almost 1 V from 10 to 100 MHz. This means that when the input interference voltage is 1 V, the output interference voltage will be the threshold voltage . When the input interference voltage exceeds 1 V, the pulse output switches on and this is rated as the heart beat. According to Irnich et al. (1996) and (Barbaro et al. (2003), the mean value for the threshold voltage
of a pacemaker is around 1–4 mV. Here we choose a typical value of 2 mV as the threshold voltage. Figure 7.21 shows the threshold output voltage, derived from the measured data in Schenke, Fichte, and Dickmann (2007), as a function of frequency between 10 MHz and 100 MHz. It is found that the threshold output voltage is almost flat within this frequency band and can have an almost constant level of 2 mV for appropriately chosen circuit parameters.
Figure 7.21 Calculated and measured output voltages

On the other hand, using the formula in Equation 7.46, we can calculate the output voltage VO for the same input voltage as given in Schenke, Fichte, and Dickmann (2007). The results are also plotted in Figure 7.21. The calculated interference output voltage is found to agree well with the measured one, which supports the validity of the nonlinear circuit model. The circuit parameters used in the model are listed in Table 7.9.
Table 7.9 Opamp circuit parameters.
Amplification factor, A0 | 1 000 000 |
Bias current, I0 (μA) | 10 |
Transfer conductance, gm (mS) | 1.2 |
Gate-to-source capacitance, Cgs (fF) | 100 |
Parasitic capacitance, CT (pF) | 1 |
This approach is applied to a HBC scenario as shown in Figure 7.22. In this scenario the pacemaker user has an on-body HBC transmitter on the chest. The transmitter is designed to communicate with the receiver at the finger position. The human body model is a homogeneous one with dielectric properties two-thirds of muscle. The on-body transmitter is an electrode structure consisting of two metal plates on the human body surface. Since the transmitter is on the chest surface, the EMI voltage produced should have a significant effect on the implanted pacemaker. Figure 7.23 shows the FDTD-calculated open voltage as a function of frequency from 10 to 100 MHz for an exciting voltage of 10 V at the transmitter. This exciting voltage should be the maximum possible level in normal on-body HBC applications. As can be seen, the produced interference input voltage decreases with frequency, which may be attributed to the more difficult penetration into the body tissue at higher frequencies. Within the interested frequency band, the open voltage
ranges from 0.18 to 0.09 V. These voltages are added to the sensing circuit input as
approximately. Figure 7.24 shows the predicted output voltage of the analog sensing circuit using the nonlinear opamp model, that is, Equation 7.46. It is found that the output voltage ranges from 0.07 to 0.02 mV within this frequency band. Compared with the sensing threshold
of 2 mV, there is a safety margin of at least 30 dB between 10 MHz and 100 MHz. This result suggests that it is unlikely to malfunction for the pacemaker under usual transmitting voltage level in HBC applications.
Figure 7.22 (a) A realistic-shaped human body model with a transmitter on the left-chest surface and (b) an implanted pacemaker inside the chest

Figure 7.23 FDTD-calculated interference voltage at the pacemaker connector. (This voltage is almost equal to the input voltage
)

Figure 7.24 Calculated output interference voltage at the pacemaker circuit in an on-body HBC scenario

7.3.4.2 UWB Pulse Signal
IR-UWB transmission makes use of short pulses to produce wide band frequency occupancy. In on-body UWB communication scenarios, communication devices on the left chest area may couple with the implanted pacemaker due to the opamp nonlinearity. In this application scenario, similar to Figure 7.22, the pacemaker user is assumed to have an on-body UWB transmitter on the chest for some biomedical purpose. Since the transmitter is on the chest surface, the EMI voltage produced may have a significant effect on the implanted pacemaker.
The UWB pulse is generally generated in the form of an nth-derivative Gaussian pulse. The expression of the nth-derivative Gaussian pulse has been given in Equation 4.5 and the corresponding Fourier transform is given in Equation 4.6. Then we have in Equation 7.21. For the EMI evaluation, we choose a second-derivative Gaussian pulse as the transmitted UWB pulse. The pulse peak is kept at 0.3 V and the pulse shape factor has been adjusted to
so as to have a spectrum close to the FCC emission requirement as much as possible. The pulse width is about 280 ps as shown in Figure 4.3.
In this analysis, the implanted pacemaker is at a depth of about 1.5 cm from the left-chest surface. Since the pacemaker can be considered as a receiving antenna, we can use the frequency-dependent FDTD method to calculate the produced voltage at the pacemaker connector as the input to the sensing circuit. On the other hand, as another alternative, based on the path loss analysis result in Section 4.2.3, the UWB pulse will undergo a 54 dB attenuation between the transmitter on the left-chest surface and the implanted pacemaker location. In view of the fact that the transmitted UWB pulse peak is 0.3 V under the FCC emission limit, we can then determine the peak voltage produced at the input of the pacemaker to be 0.6 mV. This voltage will be the maximum UWB input voltage at the sensing circuit of the implanted pacemaker.
Based on the general expression in Equation 7.21, the interference output voltage is no longer a DC component for a wideband input signal. The output voltage is calculated using Equation 7.21 and the opamp circuit parameters in Table 7.9. The result is shown in Figure 7.25. It can be noted that the voltage waveform is smoothed which is attributed to the decreased high-frequency components. Compared with the sensing threshold voltage , the peak value of the interference output voltage is only 0.037 mV. This corresponds to a safety margin of 35 dB for the pacemaker circuit for UWB interference signals. The DC component of the interference output voltage is only 0.06 μV, much smaller than the sensing threshold voltage.
Figure 7.25 Calculated interference output voltage waveform of the pacemaker circuit for maximum permissible UWB pulse input

Figure 7.26 shows the relationship between the input and output voltages for the peak voltage and DC voltage components, respectively. It can be concluded that when the peak voltage of the input pulse is less than 1 mV, both the peak and DC voltage components of the output pulse have linear relationships with the peak voltage of the input pulse. This is reasonable since when the input voltage is very small, the second term in Equation 7.21 which contains a quadratic of the input signal will become extremely small. Moreover, the frequency domain second-order Volterra kernel is much smaller than the first-order Volterra kernel
at the UWB frequency band. Therefore the nonlinear effect on input–output voltage is negligible and a linear relationship can be maintained as shown in Figure 7.26.
Figure 7.26 (a) Interference output voltage peak and (b) DC component versus input pulse voltage peak
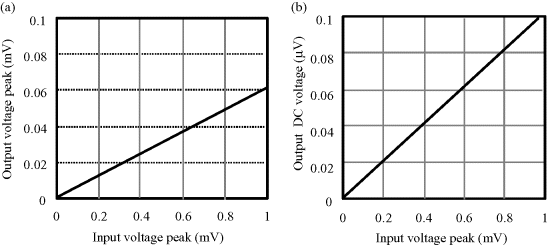
However, the demodulating property of the opamp changes the output spectral components. The interference output spectrum is not proportional to the input spectrum within the interested frequency band because of the nonlinearity. Low frequency components will be amplified while high frequency components will be attenuated. The transmission characteristics at different frequencies are shown in Figure 7.27 using the spectral amplitude ratio of output to input voltages. The high frequency components in the input voltage are demodulated and then significantly contribute to the low frequency components of the output voltage. Figure 7.28 shows the spectral amplitude ratio of the output to input voltage at 1 kHz (the cut-off frequency of the opamp) with the peak of input pulse voltage ranging from 10 μV to 1 V. The spectral component ratio at 1 kHz is as high as 230 dB which indicates a significant amplification of low frequency components. The high gain of the low frequency component is far beyond the opamp amplification ability. It should be attributed to the demodulating property of the opamp. The same result and conclusion can be drawn around the heartbeat frequency of 0.1 kHz.
Figure 7.27 Spectral amplitude ratio of interference output to input voltages
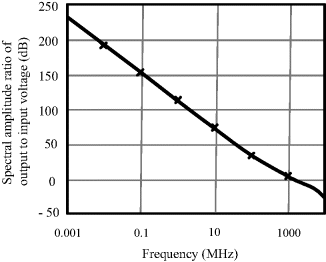
Figure 7.28 Spectral amplitude ratio of output to input voltages at 1 kHz versus input pulse peak voltage
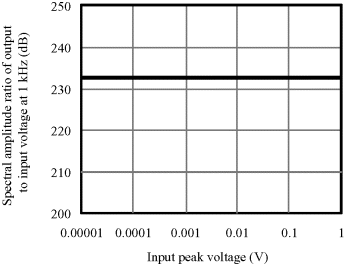
Although the above analysis has not considered the nonideal behavior of the resistors and capacitors in Figure 7.20 and has also assumed the constant parameters of opamp up to GHz frequencies, the derived finding is helpful to understand the basic mechanism and get a preliminary EMI evaluation for cardiac pacemakers. In conclusion, the mechanism of cardiac pacemaker malfunction in body area communications is mainly due to the nonlinearity of the internal pacemaker circuit. The DC and low frequency components resulting from the demodulation of the body area communication signals should be paid much attention and may be used as an index for EMI evaluation. Except for the analytical approach demonstrated above, if we have a nonlinear electric circuit model, we can also use a circuit simulator such as SPICE to derive the DC or low frequency interference voltage.
7.3.5 Experimental Assessment System
In view of the importance of EMI to the cardiac pacemaker, experimental assessment is desirable for various pacemaker products. An in vitro test system has been developed for EMI assessment of the pacemaker by mobile phones and RFIDs (Irnich et al., 1996; Tarusawa et al., 2005; Futatsumori et al., 2009). Its application to EMI assessment of the pacemaker in body area communications should be straightforward.
Figure 7.29 shows the configuration of the EMI test system for a cardiac pacemaker. The test system is composed of an electromagnetic wave source, an ECG signal generator, an ECG signal detector and a human torso phantom. The electromagnetic wave source may be a body area transceiver or a signal generator plus antenna to produce a body area communication signal. The ECG generator provides a simulated cardiac pulse to the pacemaker through an atrial or ventricle electrode. The ECG detector consists of an oscilloscope and a chart recorder. The oscilloscope is used to measure pace pulses generated from the pacemaker. The pace pulses are fed into the oscilloscope with 1 MΩ input impedance through the electrode for the atrium or ventricle, and is monitored by using a recorder equipped with sufficient digital memory to store the data.
Figure 7.29 Configuration of test system for EMI assessment of a cardiac pacemaker. (Modified from Tarusawa et al., 2005). Reproduced with permission from Tarusawa Y., Ohshita K., Suzuki Y., Nojima T. and Toyoshima T., “Experimental estimation of EMI from cellular base-station antennas on implantable cardiac pacemakers,” IEEE Transactions on Electromagnetic Compatibility, 47, 4, 938–950, 2005. © 2005 IEEE
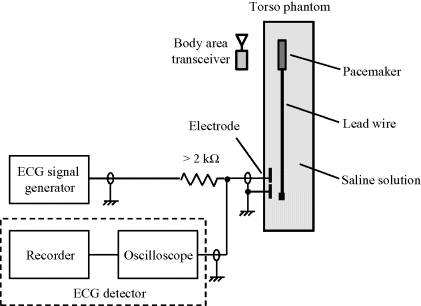
The human torso phantom consists of a saline tank and electrodes. The saline tank is constructed from acrylic boards and is filled with a saline solution (NaCl 1.8 g/l). The saline solution provides similar dielectric properties as those of the human body. The positions of the pacemaker and the lead wires are fixed by using acrylic stays in the saline tank. The size of the saline tank is adjustable to some extent. Figure 7.30 shows a detailed human torso phantom saline tank with dimensions of 34 × 36 × 3.5 cm. As can be seen, two electrodes are installed in the saline tank as either a unipolar pacemaker or bipolar pacemaker equipped with atrial and ventricle lead wires. The electrodes are constructed from 3-cm diameter stainless steel rings and 0.6-cm diameter stainless steel patches. They are used to introduce a simulated ECG signal into the pacemaker and receive the pace pulses generated from the pacemaker. Moreover, they are adjusted to isolate each chamber's ECG signal level by more than 20 dB.
Figure 7.30 Human torso model used in the test system. Tarusawa et al., 2005
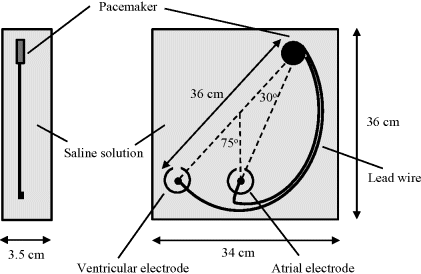
The test procedure for the pacemaker EMI, shown in Figure 7.31, consists of five steps:
Figure 7.32 shows two examples of EMI occurrence in the inhibition test and asynchronous test situations, respectively. The level of interference signal is set to be much higher than that of usual body area communication signals in order to observe an obvious EMI with the pacemaker. As a result, two inhibited pacing pulse durations are observed in (a), and three exhibited pulses are observed in (b). Compared with the analytical and numerical assessments, such an experimental approach provides a more reliable and more intuitive EMI assessment for cardiac pacemakers.
Figure 7.31 Procedure for testing pacemaker EMI
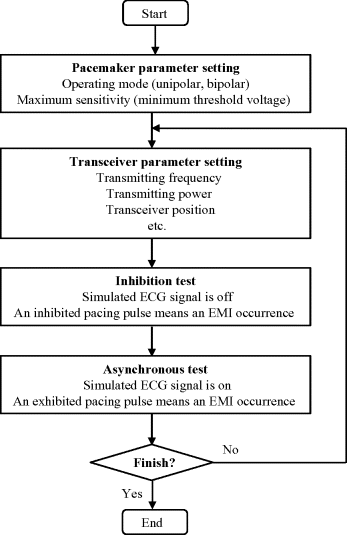
Figure 7.32 Example of EMI test results in (a) inhibition test and (b) asynchronous test
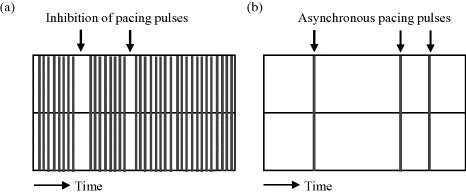
Barbaro, V., Bartolini, P., Censi, F. et al. (2003) On the mechanisms of interference between mobile phones and pacemakers: parasitic demodulation of GSM signal by the sensing amplifier. Physics in Medicine and Biology, 48, 1661–1671.
Caputa, K., Okoniewski, M., and Stuchly, M.A. (1999) An algorithm for computations of the power deposition in human tissue. IEEE Antennas and Propagation Magazine, 41 (4), 102–107.
Fiori, F. and Crovetti, P.S. (2003) Prediction of EMI effects in operational amplifier by a two-input Volterra series model. IEE Proceeding of Circuits Devices System, 150 (3), 185–193.
Futatsumori, S., Kawamura, Y., Hikage, T. et al. (2009) In vitro assessment of electromagnetic interference due to low-band RFID reader/writers on active implantable medical devices. Journal of Arrhythmia, 25 (3), 142–152.
Hartsgrove, G., Kraszewski, A., and Surowiec, A. (1987) Simulated biological materials for electromagnetic radiation absorption studies. Bioelectromagnetics, 8, 29–36.
ICNIRP (1998) Guidelines for limiting exposure to time-varying electric, magnetic and electromagnetic fields (up to 300 GHz). Health Physics, 74, 494–522.
IEEE (2002) IEEE recommended practice for measurements and computations of radio frequency electromagnetic fields with respect to human exposure to such fields, 100 kHz–300 GHz. IEEE Std C95.3-2002.
Irnich, W., Batz, L., Muller, R., and Tobisch, R. (1996) Electromagnetic interference of pacemakers by mobile phones. PACE, 19, 1431–1446.
Ito, K., Furuya, K., Okano, Y., and Hamada, L. (1998) Development and the characteristics of a biological tissue-equivalent phantom for microwaves (in Japanese). Transactions on IEICE, J81-B-I, 1126–1135.
Lin, C. (1978) Microwave Auditory Effects and Applications, Charles C. Thomas, Springfield, IL.
Schenke, S., Fichte, L.O., and Dickmann, S. (2007) EMC modeling of cardiac pacemakers. Proceedings of the 2007 International Zurich Symposium on Electromagnetic Compatibility, Munich, Germany.
Schetzen, M. (1980) The Volterra and Wiener Theories of Nonlinear Systems, John Wiley & Sons, Ltd, New York.
Schmid, T., Egger, O., and Kuster, N. (1996) Automated E-field scanning system for dosimetric assessments. IEEE Transactions on Microwave Theory and Techniques, 44, 105–113.
Tarusawa, Y., Ohshita, K., Suzuki, Y. et al. (2005) Experimental estimation of EMI from cellular base-station antennas on implantable cardiac pacemakers. IEEE Transactions on Electromagnetic Compatibility, 47 (4), 938–950.
Wang, J., Fujiwara, O., and Nojima, T. (2000) A model for predicting electromagnetic interference of implanted cardiac pacemaker by mobile telephones. IEEE Transactions on Microwave Theory and Techniques, 48 (11), 2121–2125.
Wang, Q. and Wang, J. (2009) SA and SAR analysis for wearable UWB body area applications. IEICE Transactions on Communications, E92-B (2), 425–430.
Wang, Q., Sanpei, T., Wang, J., and Plettemeier, D. (2009) EMI modeling for cardiac pacemaker in human body communication. Proceeding of the 2009 International Symposium on Electromagnetic Compatibility, Kyoto, Japan, pp. 629–632.