Chapter 5
Fuel Consumption
Fuel efficiency and emissions have become extremely important issues in the design of automobiles in order to reduce petroleum consumption and emissions that result in greenhouse effect and global warming. Overall, there are three phases involved in the conversion of fossil fuels to the delivery of useful work in controlling the motion of the vehicle in typical driving conditions. Two of these were introduced and discussed in Chapter 1 and described as:
- well-to-tank (see Figure 1.1a); Figure 1.1
- tank-to-wheels (see Figure 1.1b)
The third phase involves the conversion of power delivered at the vehicle wheel into useful work to power the vehicle over its range of normal operating conditions. Further energy losses are associated with this phase, in particular, due to tyre rolling resistances, aerodynamic drag and friction of the non-driven rotating components. Analysis of the vehicle powertrain system in typical driving scenarios depends heavily on the details of the driving schedule, involving grades, stop-start progress, traffic, acceleration and deceleration phases, etc. Consequently, there has been a lot of interest worldwide in developing standard driving cycles. These claim to be representative of typical driving conditions in different countries in, for example, urban, mixed urban, highway conditions, and they provide reference conditions so that different vehicles can be compared on a fair basis.
This chapter focuses on the second and third phases – and in particular on the calculation of the fuel consumption of the vehicle in normal driving situations. The fuel consumption of a vehicle is controlled by two main factors, namely the operating loads and the engine efficiency. The former depends on how the vehicle is used whereas the latter depends how the fuel is transformed into the work within the engine, which is often described by an engine fuel efficiency map. The loads on the vehicle vary with acceleration and climbing grades, air resistance, and the rolling resistance of the tyres. Improving efficiency, therefore, involves reducing the loads on the vehicle by proper management of vehicle performance and motion, as well as improving the efficiency of the whole powertrain and its components. Operation of the engine at peak efficiency points involves analysis of the powertrain system to ensure that the transmission is well matched to the prime mover.
The aim of this chapter is to introduce the fundamentals of vehicle fuel consumption and its calculation during normal driving. It starts with engine's fuel consumption characteristics and the dependence of fuel consumption on the engine's operating conditions. Vehicle driving cycles are universally used for standard measurements of vehicle fuel consumption and emissions, and example calculations are presented. The effect of gearbox shifting on engine working points when driving in different cycles is described and methods of automated gearshifts and their policies in fuel consumption reduction are also discussed.
The detailed energy losses in an IC engine have already been described in previous chapters – they include combustion processes, heat loss processes, pumping losses, power to drive ancillaries such as alternator, air conditioner and steering pump, etc. From the point of view of fuel consumption calculations, an overall summary of the engine properties is required. This information can be presented in several ways to quantify the fuel consumption at, for example, different engine speeds, torques and throttle conditions. The most common technique to summarize the overall engine fuel efficiency is the brake specific fuel consumption (BSFC) map.
5.2.1 BSFC Maps
The brake specific fuel consumption (BSFC) of an engine is a measure of the fuel consumption under a certain working condition. It is defined as an instantaneous ratio:
where:
(5.2)
in which m is the instantaneous mass of the fuel, and T and ω are the engine torque and speed respectively. Power Pe in Equations (5.1) and (5.3) is the brake engine power which is the mechanical output power at the crankshaft.
Using SI units, Equation (5.1) results in kg/J (kilogram per joule), however, in practice, units of grams per kilowatt-hour (kWh) are used. Conversion from kg/J to g/kWh is:
(5.4)
The conversion from metric to US customary units can be obtained from:
(5.5)
An engine operating point (EOP) can be indicated in the engine torque-speed diagram by a specific point according to the values of torque and speed, as illustrated in Figure 5.1. For example, the point EOP1 results from a throttle input of θ1 at output power P1 with torque T1 and speed ω1. Each EOP has its own BSFC value according to the working condition of the engine.
Figure 5.1 An engine operating point (EOP)

If load on the engine varies, the operating point will vary and, as a result, in the torque–speed chart, an infinite number of EOPs exist for all possible working conditions of the engine. For each point on the chart a corresponding BSFC value will be available. It happens that several of EOPs in the torque–speed plane take identical BSFC values. If these equal BSFC points are connected to each other, they will generate a curve that is a locus for a specific BSFC value. This can be repeated for all other similar points and eventually loci of constant BSFC points or iso-BSFC points result. This is called the BSFC map of an engine and a schematic map of this kind is shown in Figure 5.2.
Figure 5.2 A schematic BSFC map
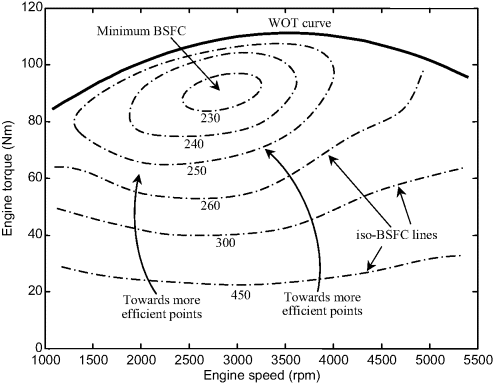
Specifying fuel consumption by the BSFC figure has a big advantage in that the BSFC values over a wide range of engine sizes remain similar. The reason is that BSFC is largely dependent on engine design factors such as compression ratio and fuel type. Therefore, for a family of engines with similar fuel type and compression ratios, the overall values for BSFC will be similar. Diesel engines have smaller BSFC values compared to petrol engines.
5.2.2 BSFC and Engine Efficiency
The engine fuel efficiency is defined as the ratio of energy produced by the engine Ee to the fuel energy supplied to that engine Ef, i.e.:
Any fuel has an energy density or specific energy defined by the fuel energy divided by its mass:
It is determined in a standardized test procedure in which a known mass of fuel is fully burned with air, and the thermal energy released by the combustion process is absorbed by a calorimeter as the combustion products cool down to their original temperature.
In order to relate the engine efficiency to BSFC, Equation (5.1) can be rewritten in the following form:
Integrating Equation (5.8) will require the time history of BSFC together with the instantaneous power of engine. If at a particular period of time or under a particular working condition, the BSFC value remains unchanged, say BSFC*, then for this particular case one finds:
(5.9)
Substituting from Equation (5.6) gives:
Combining Equations (5.10) and (5.7) gives:
Equation (5.11) indicates that the engine efficiency is inversely proportional to the BSFC and fuel specific energy values. It also shows that the engine efficiency is not a constant value and depends on the engine working conditions and in fact on the instantaneous BSFC values.
Efs basically has units of J/kg, but for Equation (5.11), it is convenient to convert Efs to kWh/g by multiplying it by 2.78×10−10.
Example 5.2.1
An engine is running at a steady condition in which the BSFC is 350 g/kWh. For a fuel specific energy of 0.0122 kWh/g, determine the engine efficiency.
Example 5.2.2
The minimum BSFC values for two typical gasoline and diesel engines together with their specific energies are given in Table 5.1. Compare the maximum efficiencies for the two typical engines.
Table 5.1 Typical engine specifications for Example 5.2.2
Engine type | Minimum BSFC ![]() |
Specific energy ![]() |
Diesel | 200 | 43 |
Petrol | 225 | 44 |
The fuel consumption or emissions for an engine depends on the way an engine is operated, which in turn depends on the vehicle operation. The vehicle operation itself is dependent on several factors such as driving pattern, gearshifts and road loads. Suppose two engine designs for a vehicle are available and designer wants to compare the performance of the two engines in terms of fuel economy and emissions when installed on a specific vehicle. Obviously both engines must be tested in similar fashion through a comprehensive test procedure so they can be compared. Driving cycles have been designed so that all test vehicles follow identical driving patterns in order that the test results for fuel consumption and pollution can be comparable.
A driving cycle is a speed-time history of vehicle motion designed to represent a real-world driving pattern. Cycle time lengths are different and could range up to 2000 seconds or more depending on the test purposes. The designs of driving cycles are based on different data collection methods [1], including realistic driving sequences that represent the normal operation of the vehicle. The driving cycles are intended to provide a compromise between real driving conditions and the repeatable measurements in the laboratory scale, with an acceptable accuracy.
Engine performance is highly dependent on geographical, cultural and legislation factors (see Figure 5.3). It is greatly influenced by the climatic variations (see Section 2.8.3). Different global regions have various climatic conditions in terms of air pressure, temperature and humidity. Road loads are different in various areas due to topographic differences. Some areas are flat and others are mountainous, thus the gravitational forces play different roles in exerting loads on engines. Driving habits in different countries also vary due to cultural, economic and traffic regulations. Local fuel prices can also affect driving styles. Average age and technology of the automobiles on the road are two other factors that create differences in the fuel consumption comparisons. The quality of fuels also plays a role in the fuel consumption and pollution generation. For all these reasons, the driving cycles for different global regions should vary if realistic estimations and comparisons are to be made. Driving cycle construction methods are different although the objectives are the same. Several countries have designed their own driving cycles based on regional influential factors.
Figure 5.3 Different influential parameters on the fuel consumption and pollution of vehicles

5.3.1 Typical Driving Cycles
Over the past two decades several test procedures have been developed and standardized by different countries in various global regions. The most common test procedure in Europe for light duty vehicle type approval is the New European Driving Cycle (NEDC), shown in Figure 5.4. The driving cycle consists of two parts, ECE15 and EUDC, that correspond to urban and highway driving conditions. The ECE15 test cycle simulates an approximate 4 km urban trip at an average speed of 18.7 km/h and at a maximum speed of 50 km/h. Its duration is 780 seconds. The same part of the ECE15 driving cycle is repeated four times to obtain an adequate driving distance and engine temperature. The EUDC cycle represents a more aggressive cycle with some high speed driving at a maximum speed of 120 km/h. Its duration is 400 seconds at an average speed of 62.6 km/h and 7 km distance. Since the year 2000, the 40 seconds idle period at the beginning of the European driving cycle (EC2000) has been omitted. Thus, the emissions during the cold start are included in the driving cycle and this makes NEDC a more realistic test procedure.
Figure 5.4 New European driving cycle (NEDC)

The FTP (Federal Test Procedure) established by the U.S. Environmental Protection Agency (EPA) is another example of a driving cycle, shown in Figure 5.5. The FTP test cycle, simulates 11.4 miles (17.7 kilometres) of driving at an average speed of 21.2 mph (34.1 km/h). The test involves a cold start after the engine's idle period, a hot start, and a combination of urban and highway conditions. The applicability of the standard driving cycles to the real-world driving patterns is also debatable. There have been efforts to design new driving cycles which claim to be more representative of real-world driving behaviour [2–4].
Figure 5.5 FTP-75 driving cycle

5.3.2 Calculations
Driving cycles can be compared based on the statistical information available. Despite the fact that only speed versus time is given in a driving cycle, from rather simple calculations, other important motion parameters such as acceleration and travel distance can be obtained, for example:
Thus from a given driving cycle, the variation of acceleration a(t) and distance S(t) can be produced by the differentiation and the integration of vehicle speed respectively. Other useful parameters can also be calculated, such as:
- average speed
- average acceleration
- total time
- maximum speed
- maximum acceleration
- other statistical measures.
Once the acceleration and distance are obtained from the speed variations (Equations (5.12) and (5.13)), the average speed and average acceleration
during a cycle are:
(5.14)
in which T is the total travel time and S is the total travel distance. It should be noted that obtained from Equation (5.15) also accounts for negative accelerations due to braking. Sometimes it is useful to find the average positive acceleration
in order to get an estimation of how dynamic the driving cycle is.
Example 5.3.1
An idealized driving cycle is given in Figure 5.6:
Figure 5.6 Driving cycle of Example 5.3.1
a. Plot the variation of acceleration and travel distance with time.
b. Determine average speed, average overall acceleration and average positive acceleration.
Solution
Since the speed-time profile includes only constant speed and constant acceleration parts, the calculation of acceleration simply involves obtaining the slopes at different points. The result is plotted in Figure 5.7.
Figure 5.7 Vehicle acceleration time history of Example 5.3.1
Finding the distance is also simple. The distance at every given time is the total area under the speed curve from time zero to that specific time. This can simply be done by calculating the triangular and rectangular sub-areas under the curve. The result is shown in Figure 5.8.The average speed can be calculated by dividing the total distance by the total time:
Figure 5.8 Variation of travelled distance with time of Example 5.3.1

The average acceleration is determined simply from the variation of acceleration (Figure 5.7) by summing up the area under the curve relative to zero acceleration. The result is:
The average positive acceleration takes only positive accelerations into account, thus it consists only of the first two terms of average acceleration:
5.3.3 Vehicle Tests
Test cycles are performed on chassis dynamometers and the vehicle is constrained to follow a speed-time pattern specified by the driving cycle. A driver controls the throttle in order that the vehicle speed remains as close to the specified speed as possible and in the meantime the emissions and fuel consumption data are recorded. Chassis dynamometers simulate the road loads and thus the test results can be considered as if the vehicle were driving on a real road.
Manufacturers' quoted fuel consumption figures are determined using standard laboratory tests on chassis dynamometers. These tests do not include several variables including road profile, traffic and weather conditions, driving style and vehicle speed, load and conditions. Such figures therefore cannot be exactly interpreted as the fuel consumption a vehicle might achieve under actual operating conditions, and are only reference values in order to compare with other manufacturers' figures.
Fuel consumption is a fundamental engineering measure and is useful because it is related directly to the goal of decreasing the amount of fuel required to travel a given distance. The terms fuel economy and fuel consumption are both used to classify the efficiency of how fuel is used in vehicles. These terms are defined as:
- Fuel economy is a measure of how far a vehicle will go with a given amount of fuel (e.g. per litre or gallon) and is expressed in kilometre per litre or miles per gallon (mpg). This is mostly the term used in North America.
- Fuel consumption is in fact the inverse of the fuel economy and is defined as the amount of fuel consumed in driving a given distance (e.g. 100 km or 100 miles) and is measured in litres per 100 kilometres (or gallons per 100 miles).
The fuel is the source of the energy for a vehicle and the work done by the vehicle is at the expense of the fuel consumption. Part of the energy a vehicle consumes during its travel is transformed to kinetic energy
and the rest,
is lost due to resistive forces. In mathematical form this is:
(5.16)
In conventional vehicles with no energy regeneration, even the kinetic energy gained during accelerating will be transformed into heat when a vehicle is eventually stopped. Thus the total energy consumption of the conventional vehicle is what the engine produces:
(5.17)
Only a fraction of the fuel energy, is transformed to mechanical power by the engine and the rest is lost (EL), or:
(5.18)
The engine fuel efficiency was defined as the ratio of energy produced by the engine to the energy supplied to that engine (Section 5.2.2). Energy is also lost within thevehicle driveline system (
) and the remaining energy
reaches to the wheels. Thus:
(5.19)
The overall driveline efficiency is defined as:
(5.20)
The overall efficiency of vehicle motion from fuel to wheel is therefore:
The transformation of wheel energy into vehicle energy involves the interactions between the road and the tyre. Another portion of energy is lost by the tyres through rolling resistance and slip. The portion related to the rolling resistance has been taken into consideration in the longitudinal dynamics (see Chapter 3) and that of the slip can be included in the tyre efficiency:
where:
(5.24)
in which the motion energy Ev is the energy that the vehicle gains during the motion and Ew is the energy delivered to the driving wheels during the same period. The overall efficiency of vehicle from fuel to longitudinal motion, therefore, becomes:
(5.25)
Combining Equations (5.21) and (5.22) results in:
This means if the values for the energy of motion Ev and overall efficiency are available, the fuel energy Ef can be determined readily. Knowing the caloric energy content or specific energy of a fuel Efs (joule/kg), the consumed fuel mass mf is obtained simply from Equations 5.7 and (5.26):
(5.27)
The overall efficiency is the inherent property of vehicle main components and is assumed to be available from test results. The motion energy Ev depends on the travelling pattern of the vehicle in which the time variation of speed is known. The method of determining Ev from driving time histories will be discussed in following section.
5.4.1 Map-free Fuel Consumption
If a constant efficiency for the engine is assumed, the engine efficiency map is replaced by a single average value for the engine performance. This will provide simple estimations for the vehicle fuel consumption. In general, driving cycles include various kinds of speed-time variations that can be divided into three types: zero, constant and variable acceleration regimes as shown in Figure 5.9. The dynamic equation of vehicle longitudinal motion in simple form is (see Chapter 3):
Figure 5.9 Different types of speed variations

in which FT and FR are the tractive and resistive forces acting on the vehicle and m is the vehicle mass including the effect of rotating inertias (see Section 3.9). The resistive force FR is in general dependent on speed and can be determined once the speed-time history is available. The details of evaluating Ev for each driving regime depend on the right-hand side of Equation (5.28) and will be discussed in the following sub-sections.
5.4.1.1 Zero Acceleration
The result of the application of Equation (5.28) for zero acceleration is:
(5.29)
Thus from Equation (5.23):
(5.30)
Note that the speed is also constant at this period and therefore for a time duration of Δt,
(5.31)
At a constant speed v, FR is also a constant value and the energy Ev only increases with time.
5.4.1.2 Constant Acceleration
For a constant acceleration a, the tractive force FT is:
Substituting into Equation (5.23) leads to:
in which S is the distance travelled and the integral term can be evaluated from the driving cycle information and resistive force values.
5.4.1.3 Variable Acceleration
Consider a segment in which a vehicle starts a variable acceleration from speed v0 at time and ends at time t and speed v. The fuel consumption in such a trip can be calculated based on the equations obtained earlier. To this end, substituting Equation into Equation results in:
The first term on the right is the net kinetic energy of vehicle during the motion and is obtained from the two end speeds. The second term must be determined using driving cycle information and resistive force values.
It should be noted that Equations 5.31, 5.33 and 5.34 are valid only for those segments of trips with no deceleration. The reason simply is that the kinetic energy gained during acceleration cannot be recuperated during deceleration, therefore it will be lost if the vehicle stops. For further clarification consider the vehicle trip illustrated in Figure 5.10 which comprises one acceleration segment starting from point A (origin) to point B and one deceleration segment from B to C. If Equation (5.34) is used to evaluate the energy Ev of vehicle, the first term on the right-hand side vanishes since the vehicle speed at both ends are nil. This result is not acceptable since if the same equation between points A and B was evaluated first, a positive sum would be calculated and since this amount of energy will be lost during deceleration while the engine is not producing power, i.e. the throttle is closed and the engine will produce braking torque if the transmission is engaged, or will work in idle if the transmission is disengaged, the total kinetic energy corresponds only to the part with no deceleration.
Figure 5.10 A sample trip

Example 5.4.1
A trip is designed in two linear acceleration and deceleration segments shown in Figure 5.11. The vehicle weighs 1200 kg and the overall efficiency from fuel to longitudinal motion is 25%.
Figure 5.11 Trip for Example 5.4.1
Resistive forces against the vehicle motion can be estimated from equation:
If the fuel has the caloric energy content of 44 MJ/kg and density of 800 kg/m3, calculate the fuel consumption in l/100 km on this particular trip.
Solution
The motion energy Ev can be obtained from either Equations 5.33 or 5.34. The second terms of both equations are identical. The evaluation of the first term on the right-hand side of equations is straightforward. From Equation (5.33) the travelled distance can be obtained by integration (or simply the area under the curve):
The first term thus results in:
From Equation (5.34):
that is exactly the same. For the second term, the variation of speed with time must be substituted.
Thus the total energy is 0.38 MJ. The fuel mass for the trip can be calculated from Equation (5.32):
The fuel volume simply is Vf = 43.13 cc = 0.043 l.
In order to convert it to l/100 km, the total travelled distance in one cycle is needed. At the end of the cycle:
Thus for a 700 m trip 0.043 l of fuel is consumed, for 100 km the fuel consumption is 6.14 l.
5.4.2 Map-based Fuel Consumption
In practice, as discussed in Section (5.2), the engine performance varies in different working conditions. BSFC as a measure of engine fuel consumption depends on the operating condition of the engine. On the other hand, engine efficiency was related to the fuel consumption measure (BSFC) in Section (5.2), by assuming a particular working condition for an engine in which the BSFC was a constant value (Equation (5.11)). The fuel mass used to produce an amount of engine output energy Ee can be obtained from Equations (5.6) and (5.10) in the following form:
This result is valid if the BSFC can be treated as a constant value. During a brief period of vehicle motion, it would be reasonable to assume it is a constant value BSFC*. In such cases, eliminating Ee from Equation (5.35) by making use of Equations and results in:
Equation (5.36) is valid only for a short period of time in which BSFC is assumed to remain constant. Therefore, in order to make use of this result for a driving cycle, the cycle must be divided into short time periods. In this way, Equation (5.36) can be evaluated separately for each time period and the total fuel consumption during a cycle can be obtained by summing up those small segments. A 1 second period is found to be appropriate for divisions of driving cycles in terms of simplicity and accuracy. A 1 second period makes the calculations very simple since the energy of each component at every division i can be replaced by its power, i.e.:
(5.37)
Evaluation of Equation (5.36) requires the calculation of two parameters Ev and BSFC at each period. A useful equation that can be used to evaluate Ev for each division is of the form:
Thus combining Equations (5.36) and (5.38) gives:
Pe (i) is simply Te (i) times the values of that are also used to determine the BSFC (i) from the engine map. Calculation of BSFC requires the engine data at different working conditions as discussed in Section (5.2). Figure 5.12 shows the general procedure involved in the calculation of the fuel consumption at each division of the driving cycle.
Figure 5.12 Flowchart of calculating fuel consumption at each division

In order to evaluate fuel consumption, again similar acceleration regimes to those explained in Section 5.4.1 can be used, with the exception that in a short time period of 1 second, acceleration can always be regarded as either constant or zero.
5.4.2.1 Zero Acceleration
Zero acceleration or constant speed portions are present in several driving cycles. For a segment in a driving cycle with constant speed, N divisions can be made (see Figure 5.13). For division i the tractive force is obtained from Equation (5.28), then the equation of motion of the driving wheel is (see Section 3.10):
Figure 5.13 Constant speed portions of a driving cycle

The wheel torque can be written as (see Section 3.13.2):
in which n and ηD are the overall gear ratio and efficiency of driveline respectively.
The vehicle speed v is directly related to the wheel rotational speed (see Section 3.10):
in which Sx is the longitudinal slip of the driving wheel. Since v is constant, the right-hand side of Equation (5.40) vanishes and by using Equations (5.40) and 41, one simply finds:
(5.43)
Engine speed for this time division can be obtained using Equation (5.42):
With values obtained for the engine speed and torque at this particular point i, the BSFC value can be read from the engine map. Ev for this division then can be obtained from Equation (5.38) and used in Equation (5.39) to calculate the fuel mass for the ith time division.
If BSFC is given in kg/J and power in Watts, the fuel mass for division i will result in units of kg/s. However, it is a common practice to represent BSFC in g/kWh. For this reason, it is necessary to convert the result of Equation (5.39) dividing it by 3.6×106, the result will be in grams of fuel in a time span of 1 second. The fuel mass for the whole cycle is then obtained by summing up all the individual fuel masses:
(5.45)
Fuel consumption (FC) can be converted to g/100 km by dividing the fuel mass by the vehicle speed and multiplying the result by an appropriate factor. Having the density of the fuel ρf, its volume can be calculated:
In Equation (5.46), v must be in km/h, mf in g/h and fuel density in kg/m3. It is also worth noting that for all divided segments the speed is equal and, as a result, the fuel consumption will be identical for all divisions. Thus it is sufficient to calculate the fuel mass only for one single division and multiply it by the number of divisions to obtain the total fuel consumption.
Example 5.4.2
The vehicle in Example 5.4.1 is driven over a 10-second segment of a cycle with constant speed of 45 km/h. At this particular point of operation, the engine BSFC is 330 g/kWh. Driveline and tyre efficiencies are 0.95 each. Determine the fuel consumption in l/100 km.
Solution
From Equation (5.28):
Engine power can be calculated from Equation (5.38):
Fuel mass in one second of motion is (Equation (5.39)):
The total mass is therefore 3.333 grams in 10 seconds. mf in one hour is 1200 grams so:
5.4.2.2 Constant Acceleration
Constant acceleration portions may be present in some driving cycles; or if a segment of a driving cycle with variable acceleration is divided into short time spans, the acceleration in such spans can be regarded as constant (see Figure 5.14).
Figure 5.14 Constant acceleration approximation

For this case, the tractive force in division i is obtained from Equation (5.32). Since v is not constant, the right-hand side of Equation (5.40) can be derived from Equation (5.44) (a zero Sx is assumed) and by using Equations 5.40–42 results in:
(5.47)
Engine speed for division i can be obtained from Equation (5.44); however, since the vehicle speed is not constant, its average value at each division is used. The rest of the procedure is similar to that of the zero acceleration case and the fuel mass can be calculated for each division and then summed up for all segments.
5.4.3 Effect of Rotating Masses
Accelerating the vehicle body takes place not only in longitudinal direction but also involves simultaneously accelerating the wheels, axles and other rotating elements, around their rotating axes. In fact, a mass that moves with speed v and rotates with speed ω has two energy components:
(5.48)
The first term in the energy equation is for the translating motion and the second term is for the rotating motion. In the discussions so far, the second term arising from the rotation of driveline components was not considered. In order to include this effect, we refer to discussions in Section 3.9, and it will suffice to substitute the vehicle mass in Equation (5.28) with an equivalent mass meq (see Section 3.9.1):
(5.49)
where:
(5.50)
in which is the sum of all inertias rotating with speed of driving wheels,
is the sum of all inertias rotating with speed of gearbox output shaft and
is the sum of all inertias rotating with the speed of the engine.
Many approaches have been attempted to improve the fuel economy of a vehicle, including optimization of the engine performance itself such as intake and fuel injection systems, increasing volumetric efficiency, optimizing the combustion chamber, etc. With the application of these methods, the performance of the engine will be directly affected and the fuel consumption map will be modified to include more areas of fuel efficient points.
In addition to these techniques which obviously influence the function of the engine itself, it is possible to optimize the manner in which the engine is utilized during driving. Improvement of the powertrain system is a means of optimizing the engine operating regimes. Possible modifications of powertrain systems can be divided into hardware and software categories. The hardware modifications affect the structure and mechanism of the powertrain system and involve the selection of different transmission types and gear ratio designs. Software modifications include the design of gearshifting schedules to optimize shift points. This is based on controlling the time spent at the most efficient engine operation points (EOPs).
5.5.1 Effect of Shifting on EOP
Operating at a particular EOP depends on the control of the input parameters, namely the throttle (or accelerator pedal) and gear ratios. In order to observe how EOPs change when the gear is changed, the engine torque–speed map will be considered from various perspectives. Engine torque and speed are dependent on vehicle kinematics and loads. The basic equations are (see Chapter 3):
in which FT and FR are the tractive and resistive forces acting on the vehicle, and n is the overall gear ratio from engine to the driving wheels. m in Equation (5.53) is regarded as the equivalent mass including the effect of rotating inertias (see Section 3.9). It should be noted that no tyre slip was assumed in deriving Equations (5.51) and (5.52) and also ideal efficiency was considered for the drivetrain. The engine torque and speed are related by the engine characteristic equation with the general form:
The overall resistive force is speed dependent (see Section 3.4):
Thus, Equations (5.51)–(5.55) show that once the vehicle speed (and its time history) have been specified, two control parameters, namely the gear ratio n and throttle θ, will govern the engine working points. The combination of these two inputs produces various possibilities for the engine operation selected by the drivers. Schematic relations of Figure 5.15 explain the processes involved in the changing of operating points according to Equations 5.56-5.60.
Figure 5.15 Interrelations between the parameters according to Equations 5.56–5.60

The engine torque-speed map can be viewed from three different perspectives: (1) throttle variations; (2) power variations; and (3) specific fuel consumption. Figure 5.16 illustrates these three views in a single figure. Moving over solid lines shows the constant throttle case, whereas dashed lines represent constant power lines. Figure 5.16 indicates that to take the engine EOP close to a minimum fuel consumption value, only limited choices for throttle opening and engine power exist. It is also clear that low and high powers are far from efficient points.
Figure 5.16 Engine torque–speed diagram and influential parameters
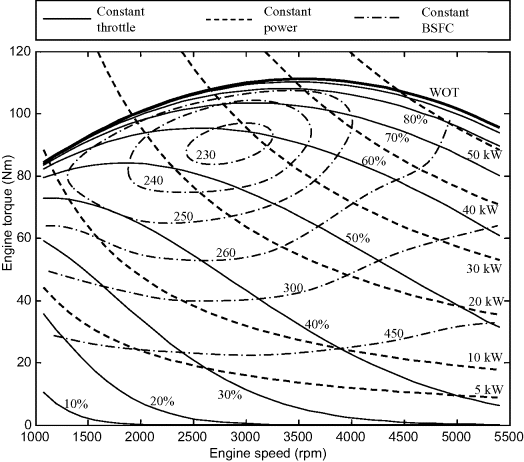
Gearshifts are carried out in order to change the EOPs, with different objectives such as:
1. Use more engine power to accelerate: this action will move EOP across the power lines.
2. Increase engine torque when more traction is required: this action will move EOP upwards.
3. Take EOP towards more efficient areas: this action will move EOP towards lower BSFC points.
4. Move away from very low and very high engine speeds: this action will move EOP towards the middle areas.
5. Use the engine braking torque: this action is usually carried out by closing the throttle completely and EOP moves downwards below the zero torque line (not shown in Figure 5.16).
All of the above-mentioned objectives can be achieved in different ways. The two available control inputs are throttle input and transmission gear ratio. If throttle input is kept unchanged during the gearshift, then the EOP will move along the constant throttle lines (Figure 5.17). Otherwise the EOP moves in any direction according to the throttle input and gear ratio.
Figure 5.17 Fixed throttle shifting
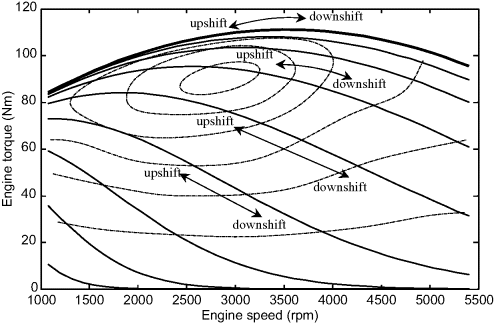
Sometimes a gearshift is performed when a constant load is acting on the vehicle. In such circumstances, the output power of engine is kept constant and the EOP is moved along the constant power lines as shown in Figure 5.18.
Figure 5.18 Constant power shifting

Example 5.5.1
The vehicle of Example 5.4.1 is moving with a speed of 90 km/h at gear 3 while the engine throttle is wide open. The engine WOT torque-speed formula is of the form:
The overall gear ratios for gear 3 and 4 are 6.86 and 4.63 respectively and the tyre rolling radius is 30 cm. If, at this instant, gear is shifted to 4:
a. Determine the new EOP and compare it with the old EOP.
b. Compare the vehicle acceleration in the two cases.
Solution
From Equation (5.51), the engine speed at gear 3 is:
Engine torque at this speed is:
When the shift to gear 4 is carried out, the engine speed and torque will change to:
Thus the EOP is moved along the WOT curve to the left since an upshift is performed. This gearshift was to move the EOP away from a high engine speed (item (4) in the list of objectives on p. 361). The vehicle acceleration for each case can be determined from Equation (5.53). The resistive force for both cases is identical:
5.5.2 Efficient Operating Points
There are several EOPs on a certain BSFC contour that apparently have similar fuel consumption values. From the definition of engine BSFC, however, it is understood that the fuel consumption is dependent on the power output of the engine as well. From Equation (5.1), the instantaneous fuel consumption can be expressed in the following form:
Equation (5.56) indicates that at a given BSFC, the instantaneous fuel consumption depends not only on the BSFC value, but also on the output power. In other words, in order to reduce the vehicle fuel consumption, it is necessary not only to direct the engine towards lower BSFC values, but also at the same time to use lower output powers.
Example 5.5.2
The vehicle in Example 5.5.1 with gearbox information given in Table 5.2 is travelling at a speed of 85 km/h. The MT formula coefficients (see Chapter 2) for the engine with the full throttle equation of Example 5.5.1 are given in Table 5.3. The minimum BSFC point for the engine is located at (T = 90, ω = 2700).
Table 5.2 Gearbox information for Example 5.5.2
Table 5.3 Coefficients of MT formula for Example 5.5.2
a. Find an ideal ratio that can bring the EOP close to the minimum BSFC point.
b. Calculate the required engine throttle for case (a).
c. Which of the actual vehicle gears is more suitable for similar dynamic performance and fuel economy?
Solution
a. From Equation (5.51) the gear ratio according to the target engine speed at the specified point is:
b. This ratio will match the vehicle speed with required engine speed. However, in order that the engine torque also coincides with the specified torque of 90 Nm, the engine throttle must be adjusted. From the MT formula with two input values of engine speed and torque, the throttle can be obtained by a trial and error procedure. The result is 56% throttle opening (see Example 4.5.5).
c. Apparently the gear ratio 3.59 is somewhere between gears 4 and 5 but closer to gear 4. In order to see how dynamically close the gears 4 and 5 are to the ideal gear ratio, the vehicle acceleration can be examined. The resistive force is:
Vehicle acceleration at the ideal gear is:
The engine speeds for gears 4 and 5 at specified vehicle speed are:
In order to produce similar acceleration at both gears, torques are obtained as (assuming no driveline loss):
Figure 5.19 illustrates the EOPs for ideal ratio (¤), 4th ratio (⊕) and 5th ratio (⊗) respectively. In terms of BSFC, the two resulting EOPs for 4th and 5th gears are located at points with not too different BSFC values, but smaller for gear 4. Since the power level for both gears is equal to 25.45 kW (and why is this?), therefore, for a similar dynamic performance gear 4 will have lower fuel consumption.
Figure 5.19 Effect of gear ratio on EOP of Example 5.5.2

A fuel efficient point on a BSFC contour is, therefore, the point with a minimum power requirement. With this concept, the Efficient Fuel Consumption Curve (EFCC), which is also called the Optimum Operating Line (OOL), is obtained from the engine torque–speed map by connecting all the fuel efficient points on different contours. In other words, at each engine power, the intersection of the constant power curve with the lowest fuel consumption contour gives a fuel efficient point for that power. Connecting all the points for different powers produces the EFCC curve. Figure 5.20 illustrates a typical EFCC curve for an engine. Tracking the EFCC in all engine output powers leads to the minimum achievable fuel consumption of the vehicle. In other words, if all EOPs could be located on the EFCC during a specific trip, all requested output powers will have been utilized with minimum BSFCs.
Figure 5.20 A typical efficient fuel consumption curve (EFCC)

Tracking the EFCC of an engine requires a gearbox with unlimited gear ratios, so that the change of transmission ratio does not lead to a sudden change of engine speed and torque. Hence, a continuously variable transmission (CVT) is required to track the EFCC with minimum error. Despite the fact that the mechanical efficiency of CVTs in general is less than that of common manual gearboxes, nevertheless this type of transmission can reduce fuel consumption by making it possible to travel smoothly along the EFCC and thus select engine operating points near to optimum working conditions by continuously changing the gear ratio.
Example 5.5.3
Suppose that the vehicle in Example 5.5.2 is equipped with a CVT gearbox. The aim is to retain the EOP at the minimum BSFC point with torque–speed specifications given previously.
For the two speeds of 70 and 100 km/h:
a. If the speeds are meant to remain constant, can the minimum BSFC point be held?
b. Calculate the gearbox ratios and throttle openings for the two given constant speeds.
c. Propose better EOPs to improve the fuel economy.
Solution
a. The answer in general is no. The reason is that when the EOP is moved over the given point, both engine torque and speed are specified. According to the discussions in Section 5.5.1 and the procedure shown in Figure 5.15, a vehicle will attain an acceleration as a result of a balance between the tractive and resistive forces. In order to have a constant speed, the tractive force must equal the resistive force at that speed. In practice, such a case can only happen accidentally.
b. The gear ratios can be determined as before by using Equation (5.51). This solution relies only on the engine speed corresponding to the specified EOP. The results for the two speeds are and
. In order to obtain the necessary throttle openings, the condition of tractive–resistive force balance must be met. The resistive forces for the two speeds are obtained as
and
. Equating the tractive forces to the resistive forces, the engine torques are found to be
and
for the two cases. The EOPs of the two cases are illustrated in Figure 5.21 (points A and B). As is clear, the EOPs are located in very poor fuel efficiency areas especially that for the 70 km/h speed. Again, using the MT formula with two input values of engine speed and torque, the throttle values can be obtained. The results are 32.6% and 40% throttle openings respectively.
c. Since the speeds are meant to remain constant, maintaining a constant power in each case will keep the traction force unchanged () which in turn does not change the speed of motion. Thus, moving the EOP over a constant power curve towards the EFCC (points C and D in Figure 5.21) can improve the fuel economy.
Figure 5.21 Effect of gear ratio on EOP of Example 5.5.3

Determining the vehicle fuel consumption in general requires the engine, the BSFC map, vehicle and driving information. Accurate calculations need additional information regarding the components' performances and efficiencies. Inclusion of driving cycles in the calculations will add to the complexity of the calculations. When engine pollution is to be determined in addition to the fuel consumption, further engine maps regarding different pollutants should also be included as inputs. All these will make the calculations more complex than one could manage without the aid of software packages.
5.6.1 Solution Methodologies
Two different methodologies are available in determining the fuel consumption and emissions of a vehicle. These are called forward-facing and backward-facing methodologies. The forward-facing methodology resembles a real vehicle's motion that involves the driver's input commands and the resulting vehicle motion. Details of such longitudinal motions of vehicle were discussed in Chapter 3. Figure 5.22 summarizes the input–output relations for a forward-facing methodology. In a forward-facing method, differential equations of motion have to be solved in order to obtain the vehicle and component states. Therefore, this type of solution is a dynamic solution in which all time-dependent phenomena are dealt with.
Figure 5.22 Forward-facing solution methodology

In the backward-facing method, as the name implies, the problem is to find ‘unknown inputs’ to the system that have led to a ‘known output’ for the system. In vehicle motion the output is typically the time history of speed (driving cycle) and the input values such as the throttle and gear ratios are calculated. A driving cycle is, therefore, the input of the backward-facing solution. Figure 5.23 shows the flowchart of a backward-facing solution.
Figure 5.23 Backward-facing solution methodology
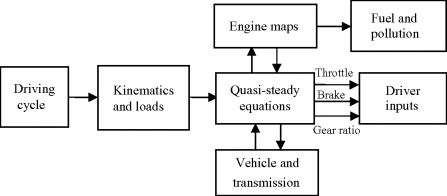
The input driving cycle is used to first determine the motion kinematics such as vehicle acceleration and travel distance as well as the components' kinematics such as rotational speeds of engine and transmission. In the next step, the loads on the vehicle and components must be evaluated so that the engine load is found. Once the engine rotational speed and load are known, the EOP is obtained and from engine performance data, the fuel consumption and emissions can be determined.
Obtaining the system kinematics and also the dynamic loads requires the differentiation of the input of the time–speed history (to obtain acceleration). A practical way to simplify this process is to divide the cycle time into small time spans and in each time span consider a constant acceleration. With this assumption, evaluation of system parameters can be carried out simply by algebraic equations (see Figure 5.12). Because efficiency maps used in backward-facing simulations are generally obtained by steady state testing, dynamic effects are not included and this is why these methods of solution are called quasi-steady solutions.
In real-world driving, if a driver wants to follow a predefined pattern like a driving cycle, the input parameters must be adjusted by receiving feedbacks from the output. In other words, the driver acts like a controller that closes the loop of the system from input to the output. Simulation in a forward-facing solution in which the input to the system is the accelerator pedal, will lead to a time history of vehicle speed but not necessarily a predefined driving cycle. In other words, in order to follow a specified driving cycle, a closed loop control system is needed to apply appropriate pedal angles at each instant so that vehicle speed versus time follows the desired pattern. In chassis dynamometer tests, a similar problem is solved since the driver must adjust the inputs according to the speed errors.
Vehicle simulators that use a forward-facing approach include a driver model, which determines the speed error as the difference between the required speed from the driving cycle input and the current speed, to estimate the appropriate throttle and brake commands so that the vehicle follows the input cycle with minimum error. Although the forward-facing solutions include all dynamic effects, backward simulations are easier to use since they do not need a closed loop control.
5.6.2 ADVISOR®
ADVISOR® is an acronym for ADvanced VehIcle SimulatOR, a simulation software package designed for the analysis of the performance and fuel economy of conventional, electric, and hybrid vehicles. The simulation environment is MATLAB® and Simulink®. ADVISOR® was designed to analyze vehicle powertrains, focusing on power flows among the components, and its main outputs are fuel consumption and vehicle exhaust emissions.
The software was first developed in 1994 by the US National Renewable Energy Laboratory (NREL) and its benchmarking began in 1995. Between 1998 and 2003, it was downloaded free of charge by more than 7,000 individuals, corporations, and universities worldwide. The input data to ADVISOR® is mostly empirical, relying on quasi-steady drivetrain component input–output relationships measured in the laboratory, and using data collected in steady state tests. The transient effects such as the rotational inertia of drivetrain components are considered in the calculations. This software was intended to be used to estimate vehicle fuel economy and investigate how conventional, hybrid, or electric vehicles use energy throughout their drivetrains. Also, it was used to determine the exhaust emissions produced on a driving cycle.
ADVISOR® is called a backward-facing vehicle simulation since the flow of information is back through the drivetrain, from tyre to axle, to gearbox, and so on. It approximates the continuous behaviour of a vehicle as a series of discrete steps. During each step the components are assumed to be in a steady state and calculations are performed at a time scale of 1 second. This assumption allows the use of power-usage or efficiency maps for the components, which are derived from steady-state tests in the laboratory. This main assumption, however, does not allow investigation of detailed, drivetrain dynamics of short duration. Examples are vibrations, electric field oscillations and similar dynamics with a time scale of less than 1 second. The input to the software is required speed (driving cycle), and it determines drivetrain torques, speeds, and powers required to meet that prescribed vehicle speed. ADVISOR® is an open source software in a MATLAB/Simulink environment and the user can modify it according to their requirements. The software was copyrighted by DOE/MRI/NREL to protect the integrity of the code because the download included the full source code, GUI, all non-proprietary data files in the ADVISOR® library, and complete documentation. However, NREL initiated the commercialization of ADVISOR® in 2003 and currently this software is no longer available in the public domain. Comments on the currently available software packages for powertrain analysis and fuel consumption calculations were included in Section 1.5 in Chapter 1.
An important subject in automatic gearshifting is designing a schedule which determines gear selection points according to a pre-defined strategy. Designers and researchers have investigated various kinds of strategies in order to reduce the fuel consumption and improve the fuel economy without sacrificing vehicle performance. Automation of gearshifts for transmission relies on decision-making algorithms depending on the vehicle's operating conditions. Gearshift decisions can be made based on two criteria: either the engine's working state or the driver's intention. The first criterion is based on moving the engine operating point towards points at which higher efficiency, lower fuel consumption or lower emissions are achieved. The engine torque and speed at any operating condition are used to determine the shift decision, with engine load and speed as two inputs similar to a conventional automatic transmission. In the second criterion, in addition to what is useful as far as the engine performance is concerned, driver's demands are also taken into account [5].
5.7.1 Engine State
Decision-making can be performed according to the engine state and load on the vehicle as is typically done in automatic transmissions. Engine life, efficiency, emissions and fuel consumption are key factors under normal operating conditions. The upper and lower speed limits of engine are limiting factors under certain working conditions. Torque capacity and output power are two governing factors at most operating points. Engine maps provide the necessary information regarding the possible movement towards optimum operating conditions and throttle value is an input element that controls the amount of available power and torque. In this case of shifts based on the engine state, only the dynamic working conditions of the engine will determine the shift decisions. To this end, a controller is needed to read the state parameters and issue a decision to maintain a gear, upshift or downshift according to the shifting strategy.
The engine load can generally be estimated according to the throttle value. The higher throttle value indicates more load on the engine and hence, more torque necessary to overcome the load. The governing laws for gearshifts, based on the engine state, can be built in accordance with an expert operator's judgement, taking into account the requirements of the engine in realistic driving conditions.
5.7.2 Driver's Intentions
The intentions of a driver are interpreted from the driver's actions during driving. Among the various parameters under the driver's control, accelerator pedal displacement and brake pedal force are the two important ones. A rather hard push on the accelerator (a large positive displacement) will mean the driver's intention is to increase acceleration and the release of the accelerator (negative displacement) will mean the opposite. Brake pedal displacements will always indicate an intention to reduce speed and eventually shift down a gear.
Gearshifts based on driver's intention need an inference system in order that the driver's actions are translated into shift decisions demands. The accelerator pedal input includes three types of information, namely:
- pedal location;
- rate of displacement;
- direction of displacement.
The output of the system is an indication of the driver's desire of how to proceed driving in terms of acceleration. The shifting controller should take all input information and issue the shift command accordingly.
5.7.3 Combined Shifting
Doing gearshifts based only on the engine state is good for fuel economy but will not necessarily satisfy the driver in terms of drivability. Shifting the gears only according to the driver's demands, on the other hand, will often make the fuel consumption worse. A compromise solution, therefore, is to take into consideration both the engine state and the driver's intention. It is, however, a complicated task to combine two contradictory requests simultaneously. The best compromise solution is to produce the power requested by the driver and at the same time try to place the EOP as close to the EFCC as possible.
5.7.4 Controller
Making decisions on gearshifts and the position of the engine operating point (EOP) is performed by a control unit. By considering a range of factors selected by the system designer (e.g. engine speed, engine load, vehicle speed, throttle angle, accelerator pedal angle and brake pedal angle, etc.) and according to the shifting strategy, the control unit determines a suitable shift action which can be to shift up a gear, to shift down a gear or no shift.
With a manual gearbox, the gearshift decision is one of the successful human control operations associated with normal driving. Although sophisticated non-linear controllers seem to work well for the decision-making of gearshifts, nevertheless the human-controlled operation is accepted as satisfactory in most cases. Thus, it is expected that a rule-based controller (e.g. a Fuzzy Logic controller) would work very well for this purpose.
The main objective for the controller could be selecting the engine operating points as close to the EFCC as possible, on various road conditions and for all inputs applied by the driver. This strategy, however, may cause behaviours unexpected by the driver such as unexpectedly increasing or decreasing the engine speed for different pedal inputs. This strategy does not take into consideration the driver's demands and only accounts for fuel economy. As stated above, in order to have a compromise, the control strategy must consider both the driver's demand and the fuel consumption at the same time. A controller based on both the engine state and the driver's intention is able to recognize the driver's intention to increase or decrease the speed and to incorporate it in the gearshift decision-making. This will in turn provide better performance using more engine power in acceleration and engine brake torque in the deceleration phases.
A controller algorithm consisting of two layers as shown in Figure 5.24 could perform the required task. In the first layer (lower layer), the outputs ‘engine state’ and ‘driver's intention’ are determined by having state inputs ‘torque’ and ‘speed’ on one hand (left), and intention inputs ‘brake’, ‘accelerator pedal position and speed’ on the other (right). In the second layer (upper layer), by receiving the two inputs ‘engine state’ and ‘driver's intention’, a controller makes the decision to shift by the commands to upshift, downshift or maintain state.
Figure 5.24 Schematic of two-layer controller operation
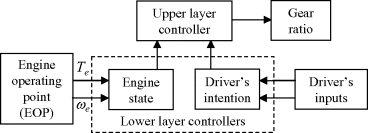
5.7.5 Multigear Transmission Concept
In Section 5.5.2, the EFCC curve was introduced and it was explained that a continuously variable transmission can be used to direct the engine to follow the curve. The question is whether it is possible to develop a geared transmission which can resemble the CVT performance. Obviously, following the EFCC exactly like a CVT is an impossible task for transmissions with finite gear ratios. Hence, a multigear transmission concept is introduced in order to make the analysis feasible, regardless of the technological barriers. Increasing the number of intermediate gears provides less difference between two adjacent ratios and in turn less amount of EOP jump during the shifts. More intermediate ratios can help the EOPs to smoothly move to a neighbouring point and the system to track the EFCC more accurately, but of course too many gear ratios need frequent shift actions. Moreover, the existence of many shift actions causes large amounts of shift delays and torque losses – both of which degrade the fuel economy. Hence, the increase of intermediate gear numbers should not exceed a certain number.
In order to find out how many gears is enough, a number of investigations were carried out using the Advisor® software and the result was that a transmission with 16 gear steps could be considered a multigear transmission. The intermediate ratios were calculated using the geometrical method (see Section 4.3.3) between the lowest and highest gear ratios. For the multigear transmission with 16 gear ratios, simulation results include the traces of EOPs that are recorded in the engine torque–speed map of Figure 5.25. The EFCC is also plotted in the same figure to make a comparison with the density of EOPs. The investigation of the trace of EOPs shows that the application of a 16-gear transmission has clearly made it possible for the points to lie close to the EFCC [6]. As the gear ratios of this virtual transmission are not as many as a CVT, EOP jumps are inevitable; nevertheless they have been placed in such a way that the EFCC curve can be regarded as the mean value of the points.
Figure 5.25 EOP placement for a 16-gear manual transmission

For the sake of comparison, the output for a five-speed manual gearbox is also generated. Figure 5.26 shows the EOP placement together with the EFCC curve. It is clear that for the five-speed transmission the EOPs are scattered at some distance away from the EFCC.
Figure 5.26 EOP placement of a five-speed annual transmission

5.8 Other Solutions for Fuel Efficiency
Obviously the process of Well-to-Wheel (see Section 1.1) is a fairly inefficient process and more work needs to be done in order to make it more acceptable. Two major approaches to increase the total efficiency of the process are related to the two main phases of Well-to-Tank and Tank-to-Wheel. Improving the Well-to-Tank efficiency is not related to the automotive industry but can be done by optimizing each portion of the upstream processes. Also substituting alternative primary energy sources instead of fossil fuels with higher energy efficiency could be useful.
Improving the Tank-to-Wheel efficiency is a bigger area for improvements since the vehicle powertrain system and especially the engine have very low fuel efficiency (see Chapter 2).
Three approaches are feasible to realize powertrain improvements. In the first approach the improvement of the efficiency of the individual powertrain components is taken into consideration. This approach has always been used by the designers and manufacturers of the powertrain components in the past and still remains one of the main challenges in the future.
The second approach is an energy management point of view that suggests making best use of components during the vehicle motion by taking advantage of the opportunities already existing in the conventional propulsion system. The third approach looks at alternative powertrain concepts that can make best use of energy sources in the vehicle and add the capability to recover the kinetic and potential energy of the vehicle that otherwise will be lost.
5.8.1 Powertrain Component Improvements
Various methods for fuel economy improvement by enhancing the efficiency of individual driveline components have been studied and employed over recent years, and researchers, designers and manufacturers will continue to tackle these issues in the years to come. These improvements can be realized in almost every aspect of vehicle design and the main items can be categorized into the three parts of weight, engine and transmission improvements. A rough estimation of the typical range of fuel consumption reduction is given in Table 5.4.
Table 5.4 Powertrain component improvement areas.
5.8.2 Lightweight Vehicles
Although making the vehicle weight lighter has been a major design goal over recent years, actually the inclusion of many comfort and safety devices has caused the vehicle weight to increase and, in future, weight reduction will continue to be one of the important issues. The direct link between vehicle weight and fuel consumption is obvious since the vehicle is accelerated and the power Pa used for this purpose simply is given in Equation 3.116:
(5.57)
in which m, a and v are the vehicle mass, acceleration and speed respectively. If the kinetic energy absorbed by the vehicle () was reversible, then a large vehicle mass would not be disadvantageous from an energy point of view. In real practice, however, this energy cannot be fully regained even in hybrid vehicles with regenerative possibility (see Chapter 7). Lightweight construction is thus an important influencing factor in reducing energy consumption. From the vehicle performance viewpoint, too, a larger vehicle mass is always disadvantageous since it results in lower attainable accelerations. Moreover, as the rolling resistance force against the vehicle motion is dependent on the mass of the vehicle (see Section 3.3), reducing the mass will in turn reduce the resistive force and improve the fuel economy.
Example 5.8.1
The mass of the vehicle of Example 5.4.1 is reduced by 10%. Consider this vehicle on the trip shown in Figure 5.11. The overall efficiency from fuel to longitudinal motion is unchanged but the rolling resistance force is reduced due to the weight reduction.
Calculate the reduction in the fuel consumption in l/100 km for this mass reduction.
Solution
The solution is similar, only the mass and the rolling resistance force must be modified. The mass is: m = 1200 × 0.9 = 1080 kg. The rolling resistance is: FRR = 200 × 0.9 = 180 N.
The result for the first term is:
The second term is:
The total energy is 0.346 MJ. The fuel mass is:
The fuel consumption is obtained as 5.63 l in 100 km, that is 10% lower than for the original vehicle. It should be noted that the linear dependency observed in this example is due to the low speeds involved and the map-free technique used.
Vehicle weight is also crucial for safety issues as in an accident a lower kinetic energy must be absorbed for a lighter vehicle. Reducing vehicle weight, therefore, is always advantageous in terms of safety, performance and fuel economy.
Lightweight design can be achieved with different methods focusing on appropriate material usage of, for example, steel, aluminium and plastics. Major technology areas are the downsizing of all possible vehicle components. Proper loading of components is another important issue since lower loads on the part will reduce its dimensions. Another area of work is X-by-wire systems in which a remote mechanical actuation is replaced by transferring a signal to the location and actuating with an electric device. Due to uncertainties of electric and electronic devices, in most cases a mechanical back-up is also necessary to ensure safety. One potential way to optimize the weight is to eliminate electric system uncertainty and remove the mechanical back-up from such systems. Optimum reduction of the weight cannot be achieved by only using lightweight and high-strength materials, but also by optimum utilization of installation space and optimum component packaging.
One more area of challenge in vehicle body design is to reduce the aerodynamic drag force (see Section 3.3) that is a resistive force in front of vehicle and consumes power. As the powertrain efficiency is improved through weight reduction, aerodynamic losses represent a greater share of total losses.
5.8.3 Engine
Despite the current trend in the direction of electric drive systems, both the spark-ignition (SI) and compression-ignition (CI) engines are still far from reaching the end of their life. This emphasizes that the efficiency of the internal combustion engine will remain critical in future vehicle applications. Losses in the engine are the single greatest source of inefficiency in vehicles. Many approaches are known to be feasible to improve the fuel economy of an engine; including the optimization of the intake and fuel injection systems, increasing the volumetric efficiency, optimizing the combustion chamber, etc. Through the application of these methods, the structure and performance of the engine will be directly affected and the fuel consumption map (Figure 5.2) will be likewise amended so that more areas of the torque-speed map are assigned to fuel-efficient points.
More improvements are needed in the part–load efficiency of IC engines as this determines the actual fuel consumption in normal driving conditions. Compared to the peak efficiency of CI or SI engines (below 40%), the part-load efficiencies are much lower on average (below 20%). Engine downsizing is important in reducing the size which will allow better packaging and weight reduction that is in line with the total vehicle weight reduction. A measure of engine sizing is its power-to-volume ratio and with downsizing a specific power output up to 70 kW per litre or even higher is now reasonable.
5.8.3.1 Gasoline Engines
Two major areas of improvement for SI engines are direct fuel injection and downsizing by using supercharging. The development potential of the SI engine is therefore considerable as the expectation of the improved combustion performance from these developments is in the order of 25% with a corresponding benefit in fuel consumption, and the resulting reduction in cylinder displacement is up to 40%. In addition, variable valve trains (VVT) and variable compression ratio (VCR) offer significant potential for fuel economy. Alternative fuels such as natural gas and hydrogen are compatible with SI engine technology and if the problems related to the infrastructure needed for using these fuels are solved, the proper combustion systems for these fuels will be easy to develop.
5.8.3.2 Diesel Engines
Modern CI engines have large power/weight ratios and their exhaust emissions have been reduced considerably over the years. In comparison to SI engines, CI engines have on average some 30% higher efficiencies and this is useful in reducing the overall fuel consumption of vehicles. Higher torque development at low rpm is another advantage of diesel engines. The downsizing of diesel engines and further reduction of friction losses will increase their potential for fuel economy. This can be done, for example, by increasing turbocharging rates, intercooling and variable valve train concepts. Advanced fuel injection systems with a variable injection-hole will reduce the exhaust emissions substantially. To increase the degree of downsizing, in-cylinder peak pressure must increase, which needs advanced supercharging and improved materials. Greater emission reduction over a wide operation in the part-load range in the engine is expected with homogeneous charge compression ignition (HCCI) without full load fuel consumption penalties.
5.8.4 Transmission
The vehicle transmission is influential in vehicle fuel economy in both hardware and software categories. Possible modifications of the transmission systems can also be divided into these two areas. First, a portion of the mechanical power generated by the engine is lost inside the transmission and not used to propel the wheels. From this viewpoint, the vehicle transmission is a power consumer and can be improved in order to become more efficient. These hardware modifications affect the structure and mechanism of the powertrain system and involve the selection of different transmission types and gear ratio designs. These types of conceptual and structural modifications of transmission system are believed to improve the fuel economy by up to 10%.
The second contribution of the transmission in vehicle fuel consumption is the way it forces the engine to operate by controlling the speed of the engine relative to the vehicle speed. In this way, it would be possible to optimize the manner in which the engine is utilized during various driving conditions. Automatic transmissions have for years accomplished this task and shifted the gears automatically in a predefined fashion.
The task of changing gears in the optimum way depends both on the hardware and software of the transmission systems. A limited number of transmission gear ratios restricts the potential for ideal adjustments of the engine operating points. In fact, the operating point of an engine jumps when a gear is changed in stepped ratio transmissions whether in manual transmission (MT) or automatic transmission (AT) modes. To be able to follow the efficient or ideal operating curve (EFCC) of an engine (see Section 5.5.2), a CVT-type transmission (see Section 4.6) is required. CVTs allow the continuous running of the engine at its optimal operating points without any interruption in power transfer. Therefore, even with ideal software programming, the optimum engine operation is not possible by using the traditional manual and automatic transmissions with a limited number of gear ratios.
On the other hand, the fuel economy is directly dependent on the efficiency of the transmission itself. Manual transmissions are simplest and are the most efficient transmissions available. Automated manual transmissions (AMT), therefore, offer the possibility for improvements in fuel economy of up to 5% owing to their high efficiency and controlling the engine operating points. These systems still have two major drawbacks of torque interruption and not being able to ideally control the engine working points. Conventional automatic transmissions have considerably lower efficiencies compared to manuals and thus their fuel economy is lower. The adoption of torque converter locks and six or more ratios, however, will mitigate the low efficiency issue.
Further possibilities are also opened up by new transmission technologies and by increasing the number of gears (six or eight speeds) as well as eliminating the torque transfer interruption. Double-clutch transmission (DCT) with seamless shifting points has significant potential for development. This technology is based on manual transmission hardware but uses two parallel transmissions for odd and even gears (see Section 4.7). This system offers the comfort of a conventional automatic transmission with the efficiency of a manual transmission and thus results in more fuel savings.
From an integrated powertrain perspective, it is important to note that the overall performance of a powertrain is dependent on matching the engine with the transmission. In practice, the engine designer should consider the shortcomings of conventional stepped ratio transmissions (automatic or manual) and include a broad speed range to allow matching to road speed. Conversely, a transmission designer should consider the operating range of the engine in determining the number of ratios in the transmission. An ideal integrated powertrain design will lead to the more efficient operation of whole system.
The fundamentals of vehicle fuel consumption evaluation were discussed in this chapter. The engine working points were shown to have different energy performances and efficiencies that are usually expressed by engine BSFC maps. There are points with higher efficiency on the engine map and operating close to these points will result in efficient driving with less fuel consumption.
Two mechanisms by which the engine operating points (EOPs) can be placed in the desired locations on the torque–speed map are the transmission gear ratios and the engine throttle opening. In each driving situation, power is demanded and for each power demand there is an efficient fuel consumption point on the map. Connecting all these points produces a curve called the efficient fuel consumption curve (EFCC). In order to obtain minimum fuel consumption, the controller should try to adjust the throttle and gear ratio in order to select the EOP close to the EFCC. A CVT was shown to be the only transmission type that can locate the EOPs over the EFCC curve. For other types of transmission with limited number of gear ratios, the EOPs cannot follow the EFCC and will be scattered around it.
It was shown that the calculation of fuel consumption for a typical driving cycle involves a large volume of information related to the engine and the vehicle that is not manageable in calculations by hand. For this reason, several software packages have been developed that provide the necessary aids for the determination of vehicle fuel consumption and exhaust pollution. It should be emphasized that even with the help of software, a large number of data inputs regarding the engine and vehicle components performances including engine maps and component efficiencies are necessary. This information must be obtained through design procedures or laboratory tests.
Further potential for the improvement of vehicle fuel economy were discussed including lightweight vehicles, engine and transmission developments. Off all the solutions, engine improvements and weight decrease have considerable further potential for reducing the fuel consumption.
5.1. Describe why the BSFC is a varying quantity during engine operation.
5.2. Where is the minimum BSFC point of an engine map?
5.3. What is the engine fuel efficiency? Can it in general be related to the BSFC?
5.4. Why are the driving cycles necessary?
5.5. What factors are the driving cycles dependent on?
5.6. Describe the difference between the map-free and map-based fuel consumption determination.
5.7. What is the basic assumption in map-based fuel consumption evaluation?
5.8. Why should the rotating masses of the vehicle be considered in fuel consumption estimation?
5.9. Describe the objectives of gearshifting.
5.10. Explain how an EFCC curve is constructed from an engine BSFC map.
5.11. Explain whether it is possible to fix the EOP of engine on the minimum BSFC point during vehicle motion.
5.12. Explain how an EFCC curve can be followed in a real vehicle.
5.13. Is it possible to obtain a good fuel economy by trying to keep the EOP as close to the minimum BSFC point as possible?
5.14. Describe forward-facing and backward-facing vehicle simulation methodologies. Which one provides more realistic outputs?
5.15. Explain the engine state and the driver's intentions in gearshifting decision-making. How can both taken into consideration in automatic gearshifts?
5.16. Describe the concept of a multigear transmission and its effect on gearshift strategy. Is it feasible to increase the number of gear ratios without limit?
5.17. What are the benefits of mass reduction in the vehicle?
5.18. The fuel consumption figures produced by an on-board trip computer are in practice different from the actual vehicle fuel consumption the driver measures. Assuming the estimation method of computer has few errors, explain the reasons for the existing differences.
Problem 5.1
Use the information given in Section 5.3.1 and show that the average speeds over the NEDC and FTP-75 cycles are almost equal.
Problem 5.2
For the NEDC cycle determine:
a. Plot the variation of acceleration with time.
b. Plot the time history of distance travelled.
c. Specify the maximum and average of vehicle acceleration.
d. Repeat (c) only for the positive values.
Results: (c) amax= 1.04 and aav= 0 m/s2; (d) amax= 1.04 and aav= 0.124 m/s2.
Problem 5.3
Repeat Example 5.4.1 for the first part of trip as a sine function of the form:
Problem 5.4
Repeat Example 5.4.1 for the driving cycle given in Figure P5.4. For the constant speed portion use the information in Example 5.4.2.
Figure P5.4 Driving cycle of Problem 5.4

Problem 5.5
Determine the map-free fuel consumption in l/100 km for a vehicle of 1500 kg mass if the driving cycle in Figure P5.5 is repeatedly used. Due to the low speeds, the driving resistance can be assumed to be a constant value of 250 N. The overall driveline efficiency from fuel caloric energy contents to traction power at the drive shafts can be assumed as a constant value of 20%. The fuel has the caloric energy content of 40 MJ/kg and density of 800 kg/m3.
Figure P5.5 Driving cycle of Problem 5.5

Result: 5.2 l/100 km.
Problem 5.6
Repeat Problem 5.5 for the driving resistance in the form of
Problem 5.7
Estimate the map-free fuel consumption in l/100 km for a vehicle with 1300 kg weight from the urban part of NEDC driving cycle. Driving resistance is and the overall efficiency can be assumed to be the constant value of 30%, from fuel caloric energy contents to traction power on the drive shafts. The fuel has the caloric energy content of 40 MJ/kg and density of 800 kg/m3.
Problem 5.8
In order to examine the effect of carrying cargo on the fuel consumption, consider the vehicle in Problem 5.5 is carrying a weight of 50 kg. How much in percentage will increase its fuel consumption? Repeat this for weights of 100 and 150 kg.
Problem 5.9
Repeat Example 5.5.2 for travelling speeds of 70 and 100 km/h.
Problem 5.10
The efficient fuel consumption curve of an engine is approximated by the equation below:
A vehicle with information given in Table P5.10 is equipped with a CVT. The vehicle is to follow the simple driving cycle given in Figure P5.10, while engine is tracking the efficient fuel consumption curve.
Table P5.10 Information for Problem 5.10.

Figure P5.10 Driving cycle of Problem 5.10

a. Ignore the low speed effects and assume that engine speed starts from 1000 rpm when the vehicle starts to move from the rest and at this moment determine the initial transmission gear ratio.
b. Determine the overall driveline ratios at the engine speeds of 1000 to 5000 rpm at increments of 50 rpm and plot the result (n versus ω).
c. Use the kinematic relation at a specified speed and calculate the transmission gear ratios at speeds of 5 to 20 m/s and plot n versus speed.
Result: (a) ng=3.74
Problem 5.11
In a micro-hybrid system the intention is to eliminate engine flywheel inertia in order to improve the fuel economy. To see the effectiveness of such concept, consider a simple driving cycle shown in Figure P5.5 and use the information in Tables P5.11.1 and P5.11.2 to find (assume no energy is lost during a gearshift):
a. total energy spent to accelerate the flywheel in one cycle;
b. average power loss (due to deceleration of flywheel);
c. fuel consumed due to flywheel dynamics in one cycle;
d. fuel consumption in l/100 km;
e. percentage of fuel economy in a vehicle with an 8 l/100 km fuel consumption.
Results: (a) 49,414 J; (b) 618 W.
Table P5.11.1 Transmission information.
Gear ratios | Max speed (km/h) | |
1st gear ratio | 3.250 | 30 |
2nd gear ratio | 1.772 | 50 |
3rd gear ratio | 1.194 | 70 |
Final drive ratio | 4.0 |
Table P5.11.2 Additional information for Problem 5.11.
Flywheel's moment of inertia | 1 | kgm2 |
Engine idle speed | 800 | rpm |
Tyre rolling radius | 30 | cm |
Fuel energy content | 40 | MJ/kg |
Fuel density | 0.8 | kg/l |
Problem 5.12
In order to examine the effect of driving aggressiveness on the vehicle fuel consumption, consider the vehicle and trip of Example 5.4.1 and increase the acceleration by having the maximum speed at 40 seconds (see Figure P5.12). Repeat this for accelerating times of 30, 20, 10 and 5 seconds and plot the variation of fuel consumption with acceleration.
Figure P5.12 Driving cycle of Problem 5.12

Problem 5.13
To study the effect of aggressive driving at higher speeds, Figure P5.13 is suggested. Use the information of Problem 5.4 and compare the increase in fuel consumption for maximum speeds of 15, 20, 25 and 30 m/s.
Figure P5.13 Driving cycle of Problem 5.13
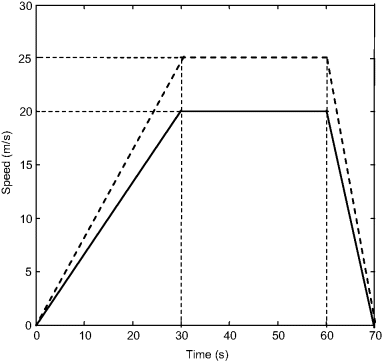
Fuel economy has always been of interest to vehicle designers – although in some markets it has until recently been of secondary importance – the focus on large ‘gas-guzzling’ engines developed in the USA for many years is an example of this. Analysis of fuel consumption features in Lucas's book [7] and he discusses vehicle design features which can be used to minimize fuel usage.
Over the past couple of decades, however, the most significant feature of vehicle design has been the need to control emissions and optimize energy usage; fortunately, these two objectives have been reasonably compatible. Emissions targets have been relentlessly driven downwards by government legislation around the world; energy usage has been driven downwards by a combination of global pressures on energy supplies coupled with consumer pressures for economic transportation. Standardized driving cycles have become a universally accepted – if controversial – method of comparing the fuel economy performance of vehicles This worldwide interest has resulted in literally thousands of journal papers and conference articles addressing the issues, but to date, very few text books. The book by Guzzella and Sciaretta [8] stands out as an excellent reference on the analysis of fuel consumption and the use of driving cycles in comparing powertrain systems.
The term ‘fuel economy’ has traditionally been associated with the usage of petrol and diesel by conventional spark-ignition (SI) or compression-ignition (CI) engines. We will return to this subject in Chapter 7 under the more general heading of ‘energy consumption’. It is central to the motivation for investigating hybrid powertrain systems that they might offer improved energy efficiency compared with conventional powertrains; hence, it is essential that we have robust and reliable techniques for analysing IC engine powertrains as a benchmark for comparisons.
There are two useful books which make a considered attempt to look forward to future developments. The textbook by Fuhs [9] is excellent reference on hybrid vehicle technology; it is up-to-date and Chapter 3 contains a comprehensive summary of all the hybrid vehicles available in 2009 – most of these are prototype developments and over 100 vehicles are described! Another particularly interesting section is Chapter 12 entitled ‘Mileage Rating’ which gives a thorough summary of testing techniques and procedures.
The book by Mitchell et al. [10] takes a much broader view of the future of personal transportation. These industry experts ‘re-imagine’ what personal transportation of the future could look like – with ‘vehicles’ that are green, smart, connected and fun to drive. Electric drive systems, wireless communication, links to infrastructure information, smart infrastructure recharging, etc. are all discussed as contributing to personal mobility in our cities. This is a fascinating and sometimes controversial text, but it certainly encourages some challenging forward thinking.
[1] Niemeier, D.A. et al. (1999) Data Collection for Driving Cycle Development: Evaluation of Data Collection Protocols. California Department of Transportation.
[2] Lina, J. and Niemeier, D.A. (2002) An Exploratory Analysis Comparing a Stochastic Driving Cycle to California's Regulatory Cycle, Atmospheric Environment, 36: 5759–5770.
[3] Andre, M. et al. (2006) Real-World European Driving Cycles, for Measuring Pollutant Emissions from High- and Low-Powered Cars, Atmospheric Environment, 40: 5944–5953.
[4] Hung, W.T. et al. (2007) Development of a Practical Driving Cycle Construction Methodology: A Case Study in Hong Kong, Transportation Research Part D, 12: 115–128.
[5] Mashadi, B., Kazemkhani, A. and Baghaei, L.R. (2007) An Automatic Gearshifting Strategy for Manual Transmissions, Proceedings of IMechE, Part I, Journal of Systems and Control Engineering, 221(15): 757– 768.
[6] Mashadi, B. and Baghaei, L.R. (2007) A Fuel Economy-Based Gearshifting Strategy. EAEC.
[7] Lucas, G.G. (1986) Road Vehicle Performance. Gordon and Breach, ISBN 0-677-21400-6.
[8] Guzzella, L. and Sciarretta, A. (2005) Vehicle Propulsion Systems: Introduction to Modelling and Optimization. Springer, ISBN 978-3-549-25195.
[9] Fuhs, A.E. (2009) Hybrid Vehicles and the Future of Personal Transportation. CRC Press, ISBN 978-1-4200-7534-2.
[10] Mitchell, W.J., Borroni-Bird, C.E. and Burns, L.D. (2010) Reinventing the Automobile: Personal Mobility for the 21st Century. MIT Press, ISBN 978-0-262-01382-6.