Chapter 1
Vehicle Powertrain Concepts
Over the past 100 years, vehicles have changed our lives; they have provided mobility which we exploit in all our commercial activities around the globe and they have also provided millions of us with new opportunities afforded by personal transportation. At the very heart of vehicle design is the powertrain system; it is the engineering of the powertrain system which provides the driving force behind the mobility.
The output from the power source – to date, dominated by the internal combustion (IC) engine – is controlled by a transmission system and driveline to deliver tractive effort to the wheels. And all these components, collectively referred to as the powertrain system, are controlled by the driver. Drivers, who are also viewed as discerning customers by the vehicle manufacturers, have a range of performance criteria: acceleration, top speed, fuel economy, gradeability, and towing capacity are some of the more obvious quantitative features. But subjective judgements such as driveability, fun to drive, refinement and driving pleasure play a huge part in the commercial success of vehicles. On the other hand, society imposes different performance demands – with a huge recent emphasis on emissions and CO2 usage of vehicles. And governments have gone as far as imposing overall emissions control targets on manufacturers' fleets of vehicles.
In order to meet all these conflicting demands, engineers must master the complete powertrain system. If there is one underlying theme to this book, it is that in order to understand vehicle mobility, one must analyze the entire system together – driver, engine, transmission, driving cycles, etc. The aim of this chapter is to provide the background to this theme.
1.1.1 Systems Approach
The key issue at the heart of this textbook is to adopt a systems approach to vehicle powertrain design. In simple terms, this means collecting all the individual components in the powertrain – or drivetrain as it is sometimes called – and analyzing how they combine and interact. The ultimate aim is, of course, to predict the overall vehicle behaviour in terms of speed, acceleration, gradeability, fuel economy, etc.
First, the behaviour of the powertrain components is analyzed – and then these components are put together as a complete system to capture the overall vehicle driveline from the prime mover, traditionally, an IC engine, through the transmission – clutch, gears, differential, etc. – to the final drive at the wheels. The important theme is that it is only by taking a system-level view of the powertrain that the vehicle designer can achieve the desired goals of vehicle performance. In a systems approach to any problem, it is important at the outset to define the system boundaries. So, for example, if we wish to study the overall use of energy in passenger car transportation, the system would look like that shown in Figure 1.1 – in which the energy is tracked from its original source through to its final usage in propelling a vehicle. This overview is important in the context of powertrain system design, and is now commonly referred to as the Well-to-Wheels analysis of energy consumption.
Figure 1.1 Overall energy conversion process in vehicle transportation – the Well-to-Wheels idea

1.1.2 History
There are lots of fascinating books describing the historical development of the automobile. It is not our intention in this book to dwell upon the history of automotive engineering; however, there are some interesting observations which set the scene for our analysis of powertrain systems.
In 1997, the SAE published an informative book [1] on the history of the automobile to celebrate its centenary. Each chapter was written by an invited US expert and all the powertrain components – powerplant (engine), transmissions, tyres, etc. – were covered. From the viewpoint of engineering innovation, it is very clear that there was plethora of innovative designs published in the late 1800s to early 1900s – but their practical exploitation was only realized decades later when material properties and mass manufacturing techniques had improved. For example, there were plenty of designs for what we consider fairly complex engineering components – automatic transmissions and continuously variable transmissions – patented in this period, but they had to wait several decades before they could be exploited commercially. The historical development of manufacturing, mass production and the economic context of automobile engineering is given in an excellent textbook by Eckerman [2].
In relation to powertrain systems, the two major components – the IC engine and the transmission have been reviewed from a historical perspective. The title of Daniels' book Driving Force [3] summarizes the role of the IC engine as the dominant power source for vehicles during the twentieth century. He presents a comprehensive overview of the detailed engineering development of engine design from its crude beginnings in 1876 as the stationary Otto engine to its current state of the art, characterized as much by sophisticated control systems as by mechanical design.
Some engineers would argue that developments in transmission design were equally important over the twentieth century. Taking the systems viewpoint, one would have to agree with this argument – since the IC engine power is only available over a limited speed range, and hence the transmission is crucial in transforming it into usable power at the wheels. Gott [4] traces the history of engineering developments in transmissions – albeit with a bias towards the US preference for automatic transmissions. This review does, however, reinforce the systems approach since the control of the transmission must be totally integrated with the engine management control.
This holistic approach, in which all the interacting parts of the powertrain are considered together, leads to the idea of system optimization. For example, while it is clearly important to optimize the design of individual components, such as the engine and transmission, the overall aim must be that they are matched together as an integrated system.
1.1.3 Conventional Powertrains
This book concentrates mainly on what are commonly referred to as conventional powertrains – in which an IC engine drives the vehicle wheels through a transmission, incorporating a gearbox and final drive unit. A typical structure for a front-engined, rear wheel drive (RWD) car is shown in Figure 1.2, with a notation of how the chapters in this book are mapped on to the powertrain system. The most common layout for small passenger cars is front-engined, front wheel drive (FWD) but the principles associated with powertrain analysis are exactly the same.
Figure 1.2 Overview of vehicle powertrain system and related book chapters

The world's total population of cars and light trucks was estimated in 2009 at around 900 million, with a production of new cars and light trucks of about 61 million in the same year. The vast majority of these – more than 99% – employ conventional powertrains as described above. Hence, despite the enormous interest from 2000 onwards in alternative powertrains, described under the general heading of Low Carbon Vehicles (LCVs), it is clear that the principles of analysing and understanding conventional powertrain systems as described in this textbook will certainly be of interest for several more decades.
1.1.4 Hybrid Powertrains
During the late 1800s and early 1900s when engineers became fascinated with the opportunities for personal transportation provided by the motor car, there were three competing technologies for the powerplant – steam, electric and petrol. Each of these had their own merits and disadvantages, and it was not at all clear at the time which was likely to dominate in the longer term. In fact, a 1900 census in the eastern US states [5] showed that each of these technologies shared about a third each of the emerging market – however, horse-drawn carriages still dominated in terms of total vehicles!
Steam had a longer history of development and there was no problem installing sufficient power to give good performance. But fuel economy was poor, the boiler needed firing up prior to a journey and both water storage and usage were problems. Electric vehicles looked extremely promising – they were quiet, clean and remarkably easy to operate. Range was the major problem limited by the available energy storage in the battery – a problem which remains to this day! Gasoline cars in that period were less well developed and appeared extremely troublesome – they were difficult to start and when running they were noisy, dirty and pretty unreliable. But their fundamental advantage – which of course is obvious now – was the energy density of gasoline which was about 300 times better than a lead-acid battery. This meant it was worthwhile investing in the engineering refinement of the gasoline-based powertrain – and this approach of relentless development and refinement has continued to the present day.
Given these discussions at the time about the best way forward for the automobile powerplant, it is not surprising that several forward-thinking engineers have suggested combining two powerplants in order to extract the benefits of each – and hence, the notion of a hybrid vehicle was born around the turn of the nineteenth century. They were not called ‘hybrid’ at the time, but it is nevertheless remarkable that, for example, the 1902 Woods gas-electric car [5] did realize the potential of what we now know as a series-electric hybrid layout. The vehicle was driven by a motor which doubled as a generator, it could run on battery power alone at low speeds, the downsized gasoline engine could be used to charge the battery, and it featured regenerative braking.
Although there are a substantial number of different powertrain architectures for hybrid vehicles, at the time of writing this book in 2011, three are of particular interest, all linked to commercially available vehicle models. These three types are summarized in Figure 1.3 and are:
(a) plug-in electric vehicle (EV), e.g. Nissan Leaf;
(b) EV with range extender, e.g. Chevrolet Volt;
(c) hybrid electric vehicle (HEV), e.g. Toyota Prius
Chapter 7 in this book introduces the highly topical subject of hybrid vehicle powertrains. It is not intended to provide a comprehensive treatment of the rapidly changing subject of hybrid vehicle technology – many excellent texts have already been written on this topic and they are referenced at the end of Chapter 7. Rather, the chapter is intended to show how the same principles of powertrain systems analysis, which are the core of this textbook, can be applied to different technologies. The aim is to show how the systems approach to the analysis of so-called conventional powertrain components can readily be applied to powertrains built up of different components such as batteries, motor-generators, fuel cells, super-capacitors, etc.
Figure 1.3 Three types of typical hybrid/electric vehicle architectures available in 2011

The components in the powertrain are described in detail in each of the following chapters in the book – and references for further reading of the best books are also provided. Needless to say, all these components are subject to relentless efforts to improve their performance – efficiency, emissions control, refinement – as well as their overall cost effectiveness. The most recent trends in powertrain component engineering are summarized below.
1.2.1 Engine
- Stratified charge combustion
- Lean burn combustion
- HCCI (homogeneous charge compression ignition) combustion
- Variable valve timing
- Supercharging or twin-charging (when coupled with a downsized engine)
- Turbocharged direct injection diesel engines
- Gasoline direct injection petrol engines
- Common rail diesel engines
- Variable geometry turbocharging.
1.2.2 Transmission
- Lower-friction lubricants (engine oil, transmission fluid, axle fluid)
- Locking torque converters in automatic transmissions to reduce slip and power losses in the converter
- Continuously variable transmission (CVT)
- Automated manual gearbox
- Dual clutch gearbox
- Increase in the number of gearbox ratios in manual or automatic gearboxes.
1.2.3 Vehicle Structure
- Reducing vehicle weight by using materials such as aluminium, fibreglass, plastic, high-strength steel and carbon fibre instead of mild steel and iron.
- Using lighter materials for moving parts such as pistons, crankshaft, gears and alloy wheels.
- Replacing tyres with low rolling resistance models.
1.2.4 Systems Operation
- Automatically shutting off engine when vehicle is stopped.
- Recapturing wasted energy while braking (regenerative braking).
- Augmenting a downsized engine with an electric drive system and battery (mild hybrid vehicles).
- Improved control of water-based cooling systems so that engines reach their efficient operating temperature sooner.
Ever since the first usable road vehicles appeared on the roads – built by, for example, Daimler, Benz, Peugeot and Panhard & Levassor in the 1890s and 1900s – people have quoted performance figures as a means of comparing vehicles. In the first instance, these were usually top speed and range; then came other performance measures as more powerful engines were installed – acceleration, gradeability and towing performance. Performance could be predicted based on very simple models using Newton's Second Law of Motion. For example, in Kerr Thomas' 1932 book [6], a chapter on the ‘Mechanics of a Moving Vehicle’ shows how to calculate speeds and accelerations based on knowledge of the engine torque and speed characteristics, gearbox ratios and estimates of the rolling resistance and aerodynamic drag terms.
According to a review paper in 1936 by the pioneering automobile engineer, Olley [7], the typical American car of that period weighed around 2 tons (2000 kg) and had an engine power of around 100 horsepower (75 kW), resulting in a typical acceleration of about 10 ft/s2 (3 m/s2), a gradeability of about 11% and a top speed around 85 m.p.h. (38 m/s
140 km/h). The accuracy of these performance predictions gradually improved from the 1930s onwards as measurement techniques for engine performance [8], tyre rolling resistance characteristics [9] and aerodynamic drag effects [10] improved. An example to illustrate approximately where all the energy is used in vehicle longitudinal performance is shown in Figure 1.4 for typical urban and highway conditions.
Figure 1.4 Example of typical energy flows during urban (a) and highway (b) driving

In the 1970s, there was a massive shift in interest in vehicle performance to focus on fuel economy calculations. In the USA, this was prompted by the Corporate Average Fuel Economy (CAFE) regulations first enacted by Congress in 1975; these were federal regulations intended to improve the average fuel economy of cars and light trucks sold in the USA in the wake of the 1973 oil crisis. Basically, it was the sales-weighted average fuel economy of a manufacturer's range of passenger cars or light trucks, manufactured for sale in the United States. This signalled the start of a huge amount of interest around the world in both fuel economy and the linked topic of emissions – and governments became very active in legislating for the measurement and control of both these aspects of vehicle performance.
In recent decades, the highly competitive commercial environment for selling cars has meant that consumers require data and performance figures to compare different manufacturers' models. Longitudinal performance – maximum speeds, acceleration, hill climbing, towing abilities, etc. – are straightforward to measure and fairly non-controversial. In contrast, however, comparative data on fuel economy, and hence emissions – have proved extremely controversial.
The established method of quantifying a vehicle's fuel economy is to subject the vehicle, mounted on an instrumented dynamometer, to a standard drive cycle. The drive cycle simply consists of a set of data points which specify a speed vs distance travelled profile. Different drive cycles have been developed to simulate different types of vehicle operation, for example, extra-urban, urban, highway, and combined urban-highway.
Although this approach is internationally accepted, substantial detailed differences have emerged in different countries and different regions of the world. Thus, global comparisons of the fuel economy of vehicles are fraught with difficulties! Broadly speaking, the current range of standard drive cycles has emerged from the world's big three automotive markets – Europe, the USA and Asia – and the differences to some extent reflect different driving patterns in those regions. An excellent overview of the comparative driving cycles is reported in [11]. The situation is further complicated by the fact that different countries or regions have developed different targets for fuel economy and emissions – which of course, makes life difficult for global manufacturers in meeting different standards for different markets.
Because of these regional differences, drive cycle testing has been a source of considerable controversy in the industry. But it has also proved extremely controversial from the consumer's point of view, because in real-world driving it has proved virtually impossible to achieve the ideal figures obtained under the standard test conditions. To the engineering community, this is an expected outcome – the tests and measurements are carried out in laboratory conditions over a repeatable drive cycle which can only be ‘typical’ of millions of real driving conditions. The key advantage is, of course, that vehicles are at least compared under fair and repeatable conditions. Nevertheless, consumer organizations and popular car publications continue to argue that the quoted figures – which now usually have to be displayed in the vehicle windscreen while on sale – should reasonably be achievable in practice.
In the European Union, the fuel economy of passenger vehicles is commonly tested using two drive cycles, referred to as ‘urban’ and ‘extra-urban’. The urban test cycle (ECE-15) was introduced in 1999 and simulates a 4 km journey at an average speed of 18.7 km/h and a maximum speed of 50 km/h. The extra-urban cycle (EUDC) simulates a mixture of urban and highway running; it lasts 400 seconds with an average speed of 62.6 km/h and a top speed of 120 km/h. In the USA, the testing procedures are administered by the Environmental Protection Agency (EPA) and were updated in 2008 to include five separate tests – which are then weighted together to give an EPA City and Highway figure that must be quoted in car sales information. It is claimed – with some justification – that these figures are a better reflection of real-world fuel economy performance than the EU figures.
Just to add to the confusion, fuel economy continues to be quoted in different units around the world. For example, both the USA and the UK use miles per gallon (mpg) – although even these are not comparable since the US gallon is 0.83 of an imperial gallon! In Europe and Asia, fuel consumption is quoted in units of l/100km. Note that both lower (l) and upper case (L) can be used for litres. This is effectively an inverse of the mpg approach and a large mpg is comparable to a small l/100km – so, for example, 30 mpg = 9.4 l/100km and 50 mpg = 5.6 l/100km.
However, most vehicle analysts agree that overall, the drive cycles are all less aggressive than typical real-world driving; in practice, this means that they include lower values of acceleration and deceleration than typically used in normal driving situations. With the upsurge of interest in hybrid powertrains over the first two decades of 2000, there has inevitably been an enormous focus on promoting their potential fuel economy relative to conventional powertrains. This has generated an on-going debate about whether the drive cycles tend to favour HEV powertrains over conventional ICE-based powertrains. The underlying principle is that HEVs offer the biggest scope for improvement under stop-start driving conditions in heavy city traffic, for example; hence, it is argued that since most drive cycles have their bias towards urban operation and inclusion of idle periods, they can distort the potential benefits available from hybrid powertrains – but again, there are a wide range of views!
In relation to emissions, there are two aspects; both of them are commonly referred to as ‘tailpipe emissions’ for the rather obvious reason that they emerge from the exhaust pipe as products of the combustion process. The first issue is the pollutant emissions – these include carbon monoxide (CO), unburnt hydrocarbons (HC) and oxides of nitrogen (NOx). In Europe, engine emission standards were introduced in the early 1990s to reduce all these pollutants from vehicles. It led to significant improvements in harmful emissions from passenger cars. Euro 5 is due to come into effect for passenger cars in 2011 and a further tightening of the regulations, Euro 6, is planned after that for both commercial vehicles and cars.
The second issue is the carbon dioxide (CO2) emission levels of vehicles. These have assumed increasing attention during the early part of the twenty-first century due to global concerns about the environment – and they form part of the carbon footprint calculations which have now become embedded in all aspects of life. In the UK from 2001, the vehicle tax was linked to the CO2 emissions of new vehicle, so that vehicle emitting less than 100g/km were actually free of road tax. And in 2008, an ambitious piece of legislation was passed which committed European car manufacturers to cut average CO2 emissions from new cars to 130g/km by 2015.
Although the focus of this textbook is entirely on the vehicle and the engineering of its powertrain system, it is important to recognize that whenever a vehicle is used on the road, the complete system actually involves both the vehicle and its driver. The complete system is shown in Figure 1.5, in which the driver effectively acts as a feedback controller – monitoring the performance of the vehicle and feeding back this information to compare with his demand signals to the accelerator, brake, gear selection, etc. Thus, from a dynamics point of view, we are in practice dealing with a control system. In designing the vehicle engineering system, therefore, we must be aware of the driver preferences as a controller.
Figure 1.5 Overview of the driver–vehicle system governing vehicle longitudinal performance
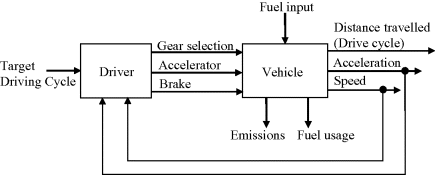
In subjective terms, drivers tend to prefer systems which are:
- responsive
- controllable
- repeatable
- stable
- involving minimum time lags
- linear
- free from jerks or sudden changes.
The study of drivers' assessments of the longitudinal control of the vehicle is called ‘driveability’ and it is emerging as a crucial feature of vehicle refinement to assess the customer acceptance of new powertrain components. For example, it has been used in the industry from 2000 onwards to assess the smoothness of gear changes in new transmissions developments such as dual clutch gearboxes and continuously variable transmissions (CVTs). Indeed, procedures for the assessment of the highly subjective perception of the driver have been incorporated into specialized vehicle software packages such as AVL-DRIVE [12]. The idea is to generate an objective measure which is based on subjective judgements made by drivers using a range of vocabulary such as – jerk, tip-in, tip-out, kick, response delay, oscillations, ripple, backlash, etc. – some of which have more obvious interpretations than others.
There are occasions in vehicle performance calculations and simulations in which it is necessary to include a mathematical model of the driver in the complete system, as shown in Figure 1.5. In the so-called ‘forward-facing’ simulation, discussed in the next section, it is necessary to have a driver model which attempts to follow the specified driving cycle by applying appropriate signals to the accelerator and brake inputs. The approach used in this case is often a simple PID (Proportional Integral Derivative) model. This is good for tracking the speed profile, but is not necessarily representative of actual driver behaviour which is likely, for example, to include some element of look-ahead preview.
The whole ethos of this book is based on a modelling approach to analyzing and understanding powertrain system design. The underlying aim is to explain how components function and then represent their behaviour through mathematical models based on the physics of their operation. Then, the components can be combined together as a complete powertrain system – and the resulting model should provide an important tool to contribute to vehicle design. Thus, although an analytical approach is used in order to understand the fundamental behaviour, the results are always aimed at being of practical value to vehicle engineers.
The models used throughout the text are relatively simple – and examples are provided in which the models are expressed and solved in the MATLAB®/SIMULINK environment. Thus, it should be easy to follow the complete process from the derivation of the governing equations, through to their coding in MATLAB/SIMULINK to their solution and presentation of results. Since the book is based on fundamental issues, it is felt to be important that the reader – whether a student or a practising engineer – can follow this whole procedure and try it out for themselves.
In calculations of vehicle performance over a specified driving cycle, there are two fundamentally different approaches – which are often not well understood by newcomers to the subject area. The most common simulation is called a ‘backwards-facing’ calculation. This means that at each point on the speed vs distance profile, the current values of both the vehicle speed and acceleration are known and using these it is possible to work backwards through the powertrain to calculate the speeds, accelerations, torques and powers of all the components. This process is simply repeated for all the points on the driving cycle and the results summed together at the end. This is the simplest and most commonly used method of predicting vehicle performance over a drive cycle.
The other approach is called a ‘forward-facing’ simulation; this requires a driver model in addition to the vehicle model. The drive cycle is a target trajectory which the driver tries to track via inputs to the vehicle system. The simulation is performed then as a conventional time history simulation, involving integration of the dynamic equations. This approach is required when developing control systems for the powertrain elements in order to simulate how the controller would actually behave in real time in the vehicle.
For more detailed analyses of powertrain components and systems, several commercial packages are available. These are used extensively in vehicle design offices around the world, and while they undoubtedly offer increased fidelity in their representation of the engineering systems involved, they are less informative of the underlying mechanics. Examples of such packages include;
- ADVISOR – (ADvanced VehIcle SimulatOR) was created by the U.S. Department of Energy's National Renewable Energy Laboratory's (NREL) Center for Transportation Technologies and Systems in 1994. It was a flexible modelling tool that rapidly assesses the performance and fuel economy of conventional, electric, hybrid, and fuel cell vehicles. It was acquired by AVL in 2003 [12].
- AVL CRUISE – Vehicle and driveline system analysis for conventional and future vehicle concepts [12].
- AVL-DRIVE – Assessment of driveability [12].
- CarSim – Vehicle performance in response to braking, steering and accelerating inputs [13].
- IPG CarMaker – Vehicle performance in response to braking, steering and accelerating inputs [14].
- Dymola – A multibody systems dynamics packages with automotive as well as other industrial applications [15].
- WAVE – 1D engine and gas dynamics simulation; also includes a drivetrain model to allow full vehicle simulation [16].
- SimDriveline – Blocks to characterize driveline components to include in a Simulink environment [17].
- Easy5 – Multi-domain modelling and simulation of dynamic physical systems [18].
The overall aim of this book is to provide a comprehensive and integrated overview of the analysis and design of vehicle powertrain systems. This has the following objectives:
- to present a summary of the systems approach to vehicle powertrain design;
- to provide information on the analysis and design of powertrain components, in particular:
internal combustion engine
transmissions
driveline components
- to analyze the longitudinal dynamics of the vehicle in order to predict performance;
- to analyze and discuss the fuel economy performance of vehicles;
- to analyze the torsional dynamics behaviour of the driveline system;
- to describe the fundamentals of hybrid electric components and the architecture of their usage in a hybrid vehicle powertrain;
- to present examples – some with worked solutions – throughout the text;
- to present case studies of powertrain performance using MATLAB as an analysis tool.
The books listed as references [1–5] all provide excellent background information on the history of automotive engineering, IC engine, transmissions and hybrid vehicle developments. They are all worth reading to set the scene for powertrain systems analysis.
[1] SAE (1997) The Automobile: A Century of Progress. SAE, ISBN 0-7680-0015-7.
[2] Eckermann, E. (2001) World History of the Automobile. SAE, ISBN 0-7680-0800-X.
[3] Daniels, J. (2003) Driving Force: The Evolution of the Car Engine. Haynes Manuals, 2nd edn, ISBN 978-1859608777.
[4] Gott, P.G. (1991) Changing Gears; The Development of the Automatic Transmission. SAE, ISBN 1-56091-099-2.
[5] Fuhs, A.E. (2009) Hybrid Vehicles and the Future of Personal Transportation. CRC Press, ISBN 978-1-4200-7534-2.
[6] Kerr Thomas, H. (1932) Automobile Engineering, Vol. 1. Sir Isaac Pitman & Sons.
[7] Olley, M. (1936) National Influences on American Passenger Car Design. Proc. Institution of Automobile Engineers, Vol. XXXII, pp. 509– 541.
[8] Plint, M.J. (2007) Engine Testing, 3rd edn. SAE International, ISBN: 978-0-7680-1850-9.
[9] Clark, S.K. (ed.) (1981) Mechanics of Pneumatic Tyres. DOT HS 805 952, U.S. Dept of Transportation.
[10] Hucho, W-H. (ed.) (1998) Aerodynamics of Road Vehicles, 4th edn. SAE International, ISBN 0-7680-0029-7.
[11] Samuel, S., Austin, L. and Morrey, D. (2002) Automotive Test Drive Cycles for Emission Measurement and Real-World Emission Levels: A Review. Proceedings of the Institution of Mechanical Engineers, Part D: Journal of Automobile Engineering, 216 (7): 555– 564.
[12] www.avl.com (last accessed March 2011).
[13] www.carsim.com (last accessed March 2011).
[14] www.ipg.de (last accessed March 2011).
[15] www.dymola.com (last accessed March 2011).
[16] www.ricardo.com (last accessed March 2011).
[17] www.mathworks.com (last accessed March 2011).
[18] www.mscsoftware.com (last accessed March 2011).