Chapter 3
Carrier Aggregation
International Mobile Telecommunications Advanced (IMT-Advanced) requirements establish a minimum support of 1 Gbps and 100 Mbps peak rates for low-mobility and high-mobility users, respectively. In order to fulfill these challenging requirements, wider channel bandwidth than legacy Third Generation (3G) systems has to be supported. However, the available spectrum resources of operators differ depending on the specific country, being spread out over different frequency bands and with different bandwidths. Therefore, all IMT-A technologies foresee one of their key features as the aggregation of continuous or discontinuous spectrum in order to achieve wider bandwidth and consequently increase transmission capability. This concept is usually known as Carrier Aggregation (CA) or spectrum aggregation.
Discontinuous CA has the advantage of having spectral diversity gain, due to the use of different frequencies, which implies different types of fading channels. However, this requires having several physical layer processing chains – one per each of the aggregated carriers. On the other hand, contiguous CA can save much spectrum because many subcarriers used as guard bands can be employed for data and control information. In the absence of carrier aggregation some frequency chunks became unused whereas with carrier aggregation these guard bands could be removed.
Moreover, contiguous carrier aggregation could use only one Base Band (BB) processing chain (large Fast Fourier Transform (FFT) block), if the transmitter and receiver are suited to it, thus eliminating the need for parallel Radio Frequency (RF) transceivers.
There are some concepts defined in the International Telecommunication Union – Radiocommunication Sector (ITU-R) regarding CA that must be clarified to understand the main research challenges and their constraints:
- Carrier Aggregation: to transmit data on multiple carriers, or sub-bands, contiguous or non-contiguously located by using one or several parallel RF transceivers. The User Equipment (UEs) may adopt a single wideband-capable RF front-end and a single FFT, or multiple legacy RF front-ends (<20 MHz) and FFT engines. The choice between single or multiple transceivers depends on power consumption, cost, size, and flexibility to support other aggregation types. Larger bandwidths than 20 MHz can be supported using carrier aggregation.
- Component Carrier (CC): the independent RF sub-band, or carrier, that is aggregated with other sub-bands to conform a larger bandwidth. Each component carrier maintains its original structure to support single-carrier-capable users, even if it is aggregated to a larger bandwidth.
- Guard Band: the guard subcarriers that cannot be used for transmission. In the case of contiguous carrier aggregation this guard band is located at the edge of the aggregated bandwidth. In case of noncontiguous aggregation, each sub-band will have its own guard bands, thus reducing efficiency.
- Center frequency of the aggregated bandwidth: the center of the total aggregated bandwidth.
- Center frequency of the component carrier.
In the IEEE 802.16m draft standard a further subclassification of component carriers is made. According to the transportation of signaling information, CCs are classified as:
- Primary Carrier: this is the carrier used by the Base Station (BS) to exchange control signals plus additional traffic data with the UE. Full control of UE mobility, state and context is made through the primary carrier. It is worth noting that each UE has only one primary carrier allocated. However, from the point of view of the BS all component carrier may act as primary carriers for some UEs.
- Secondary Carrier: one IMT-Advanced UE might have one or several secondary carriers allocated for traffic exchange. These secondary carriers are controlled by the signaling conveyed in the primary carrier.
Concerning their usage, CC are further classified into:
- Fully configured carrier: a primary carrier is always fully configured since all control channels are transmitted. A secondary carrier for a UE, in case of being fully configured, may serve as primary carrier for others UEs.
- Partially configured carrier: this CC cannot operate on a stand-alone basis because it only carries a limited set of control channels. These partially configured carriers are mainly configured for downlink-only transmissions, such as multicast/broadcast traffic.
Finally, from the UE's operation perspective, the IEEE 802.16m draft standard also defines two different multicarrier operation modes:
- Multicarrier aggregation: in which the UE receives control information from primary carrier and processes data on the primary or secondary carriers. The UE must be able to receive simultaneously data from all component carriers since the Medium Access Control (MAC) layer scheduler may assign resources to the UE in the primary and multiple secondary carriers. Therefore, channel state reporting mechanisms should inform about all active component carriers.
- Multicarrier switching: in which the UE is connected to just one component carrier at a time switching from the primary to the secondary carrier according to the control information. The UE connects with the secondary carrier during a specified time period and then comes back to the primary carrier to receive further instructions.
Dealing with CA, several research issues need to be analyzed in depth to exploit fully the opportunity offered by bandwidth increase. Some of these research topics are treated in this chapter considering the investigation from several perspectives. Section 3.2 deals with regulatory aspects. Section 3.3 makes a deep analysis of the state of the art focusing on the results derived from the Wireless World Initiative New Radio (WINNER+) project. Section 3.4 focuses on the cognitive aspects related with CA and Section 3.5 addresses signalling issues. Finally, section 3.6 discusses about hardware and legal limitations around CA concept.
3.2 ITU-R Requirements and Implementation in Standards
The usage of radio frequencies is administered globally by the ITU-R via the Radio Regulations (RR). The RR is a binding agreement between governments. In ITU-R, the world is divided into three regions and the allowed spectrum use in RR differs among those regions. Region 1 consists of Europe, the Middle East and Africa, Region 2 is North- and South America and Region 3 is Asia Pacific. ITU-R specifies the spectrum use allowed mainly by allocating spectrum bands to different radio services. A service in ITU-R means a certain type of usage, characterized for example by some transmission characteristics or a kind of transmitter. There are approximately 40 ITU-R services, for example, mobile service, fixed service and fixed satellite service. The whole radio spectrum is allocated to different ITU-R services, thus, there is not unused available spectrum. Spectrum allocations can be either primary or secondary allocations. The services with primary allocations have a higher status and priorities than the services with secondary allocations. A band usually has more than one allocation in RR from which national administrations can choose. This gives considerable freedom for national administrations to decide how to use each band. National administrations are also in charge of dividing the portions of these spectrum bands, for example, in the case of mobile communications to different operators (Takagi and Walke 2008).
ITU-R has defined several requirements for IMT-A systems. These requirements are related to technical performance, spectrum and supported services. Regarding the spectrum bands, an IMT-A system has to support a scalable bandwidth up to and including 40 MHz. This may be supported by single or multiple RF carriers. The scalability is defined as an ability to support at least three bandwidth values. As an example, the Third Generation Partnership Project (3GPP) Long Term Evolution (LTE) Release (Rel-8) system is able to support six different bandwidth values up to 20 MHz. In order for LTE Release Rel-10 (LTE-Advanced (LTE)) to meet the IMT-Advanced requirement for system bandwidth, it needs to aggregate at least two CCs to support wider transmission bandwidths effectively. Even ifITU-R has set the requirement for the supported transmission bandwidth to 40 MHz, the IMT-A systems are encouraged to support operation in wider bandwidths – even up to 100 MHz – to enable systems to reach the research target peak data rates: 100 Mbps for high mobility and 1 Gbps for low mobility 1 Gbps for low mobility (ITU-R 2008b)
Another spectrum related requirement is that IMT-Advanced systems need to be able to utilize at least one band identified for IMT citep (ITU-R 2008a). In World Administrative Radio Conference (WRCs) (World Administrative Radio Conference (WARC) in 1992, WRC in 2000 and in 2007), the following spectrum identifications for IMT-2000 have been made:
- 450–470 MHz
- 698–960 MHz
- 1710–2025 MHz
- 2110–2200 MHz
- 2300–2400 MHz
- 2500–2690 MHz
- 3400–3600 MHz
The list is simplified and the actual allocations vary considerably on different ITU-R regions. Actually, the widest contiguous spectrum allocated for IMT is 200 MHz on the 3.4-3.6 GHz band, which is available in a large number of countries in Regions 1 and 3. Therefore, the amount of contiguous transmission bandwidth for one operator in a certain geographical area is limited. In order to meet both the requirement on transmission bandwidth and the requirement for utilizing the IMT bands, the concept of CA is crucial. Therefore, CA has been identified as one of the major features in both 3GPP LTE-A and Institute of Electrical and Electronics Engineers (IEEE) 802.16m technologies to meet the IMT-Advanced requirements.
In 3GPP, three possible CA scenarios — also shown in Figure 3.1 — are considered:
- Intraband contiguous CA, where contiguous bands wider than 20 MHz are used.
- Intraband noncontiguous CA, where multiple CC belonging to the same band are used in a noncontiguous manner.
- Interband noncontiguous CA, where multiple CC belonging to different bands are aggregated.
Figure 3.1 Carrier aggregation

In the Uplink (UL), the focus is on the intraband noncontiguous case, whereas in the Downlink (DL) the interband case is also considered. Four different deployments are considered for CA. In one of the most typical deployment scenario the antennas are collocated and have the same beam patterns for different components. If the CC are at the same band or the frequency separation is small, this would lead to nearly the same coverage for all CCs. Large frequency separation between CCs would lead to the second scenario where the coverage of the CCs are different. In the third scenario, different beam directions or patterns are used for different CCs to shift the beams across carriers and by doing so improve throughput at sector boundaries. In the fourth scenario, one CCs provides macrocell coverage and remote radio head cells are placed at traffic hotspots for additional throughput by other CCs. This last scenario is, however, not considered in UL (Iwamura et al. 2010.
On the other hand, IEEE has not clearly stated, in the IMT-Advanced candidate proposal, which are their choices for carrier aggregation. Only the following text can be found in (IEEE 802.16 2010) concerning the IEEE 802.16m requirement of the operating bandwidth: “[...] a common MAC entity to control a PHY spanning over multiple frequency channels. The channels may be of different bandwidths (e.g. 5, 10 and 20 MHz) on contiguous or non-contiguous frequency bands. The channels may be of the same or different duplexing modes, for example, FDD, TDD, or a mix of bidirectional and broadcast only carriers [...].”
The challenges that arise from CA are mainly due to the fact that UEs can transmit and receive from more than one carrier – and in theory from more than one cell. This requires some changes in the radio layer 1 and 2 and adds complexity. It changes Radio Resource Management (RRM) requirements because the UE will need to measure several carriers at the same time. The UE power consumption is increased with the number of CC because UE will have to monitor more physical downlink control channels, which are detected by blind decodings. Two methods that are investigated to allow power savings are discontinuous reception and component carrier activation/deactivation. Moreover, the design and deployment of control signaling channels for multiple CCs is crucial for efficient data transmission control and the overall system performance. Because of different interference conditions on different carriers, LTE-A needs to support CC-specific UL power control for both contiguous and noncontiguous CA. Mobility management, more specifically radio resource control measurements and handovers, are also issues that require specific attention (WINNER+ 2010.
As a step from carrier aggregation towards more flexible spectrum use, cognitive radio introduces a novel approach to accessing spectrum – in an opportunistic way. This may increase the efficiency in spectrum use, however, the rights of current spectrum users need to also be preserved. The introduction of cognitive radio techniques therefore also requires acceptance from the regulatory domain. As a sign of awareness of this new emerging technology in the regulatory domain, the next WRC of ITU-R in 2012 will consider regulatory measures and their relevance in order to enable the introduction of Software Defined Radio (SDR) and Cognitive Radio System (CRS) (agenda item 1.19). The preparatory work for this agenda item at the European level in European Conference of Postal and Telecommunications Administrations (CEPT) Electronic Communications Committee (ECC) has been conducted in a Conference Preparatory Group (CPG) Project Team A (PT A), which has been responsible for all the aspects of the agenda item. The European Conference of Postal and Telecommunications Administrations (CEPT) Electronic Communications Committee (ECC) response to the agenda item finished in June 2010 and it was sent to the ITU-R Working Party (WP) 1B “Spectrum management methodologies and economic strategies,” which has the main responsibility for the preparations on this agenda item at the international level. As the first step towards the next WRC, ITU-R WP 1B developed the definitions for CRS and SDR in September 2009. The official International Telecommunication Union (ITU) definition for CRS is “[...] a radio system employing technology that allows the system to obtain knowledge of its operational and geographical environment, established policies and its internal state; to dynamically and autonomously adjust its operational parameters and protocols according to its obtained knowledge in order to achieve predefined objectives; and to learn from the results obtained [...]” (ITU-R2009a). ITU-R WP 1B has also finished its work on this agenda item in June 2010 by finalizing the Conference Preparatory Meeting (CPM) text. ITU-R WP 5A “Land mobile service excluding IMT; amateur and amateur-satellite service” has been responsible for the technical work regarding the agenda item. ITU-R WP 5A is developing an ITU-R report “Cognitive radio systems in the land mobile service.” The purpose of this report is to provide answers to the questions posed by the ITU Radiocommunication Assembly (RA) in 2007. The content of the report covers the following aspects of the CRS:
- the ITU definition;
- closely related radio technologies and their functionalities;
- key technical characteristics;
- requirements;
- performance;
- benefits;
- the potential applications;
- the operational implications;
- capabilities that facilitate coexistence with existing systems;
- possible spectrum-sharing techniques; and
- the effect on the efficient use of radio resources.
There are also various other ongoing activities regarding CRS at the European and international level. At the European level, in CEPT ECC Working Group Spectrum Engineering (SE) 43 is investigating the use of cognitive radio systems in the TV white spaces, that is, 470 - 790 MHz band. The outcome of these investigations is reported in the ECC report “Technical and operational requirements for the operation of cognitive radio systems in the white spaces of the frequency band 470–790 MHz.” The report is mainly band specific, considering sharing scenarios and national deployment scenarios of systems or services to be protected. However, some of the issues may be applicable for other frequency bands. CEPT ECC Working Group Frequency Management (FM) has initiated work to monitor and investigate the Cognitive Radio (CR) technological and characteristics development and to study how to regulate the spectrum requirements of such technologies. The working group will receive information by monitoring the European Union research projects and the European Telecommunications Standards Institute (ETSI). At the international level, ITU-R WP 5D “IMT Systems” is also working on CRS and the outcome of their studies will be published in a report “Cognitive Radio Systems Specific for International Mobile Telecommunications (IMT) Systems.”
3.3 Evolution Towards Future Technologies
The purpose of this section is not to clear up the current technology of spectrum aggregation in the future Fourth Generation (4G) standards of mobile communications such as LTE-A or Worldwide Interoperability for Microwave Access (WiMAX) IEEE 802.16m, but to present novel techniques, algorithms or proposals based on spectrum aggregation that could be employed in those future standards. Even though this section mainly focuses on LTE-A technology, some proposals could be used in future releases of WiMAX.
With the arrival of CA, a large number of open issues should be addressed in the future. Is it possible to use a different coding method that improves the performance with longer block sizes? Is it better to schedule resource in a contiguous or noncontiguous way? Which are current trends concerning scheduling in IMT-A systems? What is the best reporting process in CA? Finally, this section presents current trends in CA for IMT-Advanced systems.
3.3.1 Channel Coding
Obviously, if larger bandwidths are used then transmission capability generally increases. Nevertheless, when a BS has to transmit data to several frequency bands the best choice is still not clear whether to encode data in separate transport blocks or to combine all information and distribute the bits in the physical layer. In the first case each CC has its own configuration parameters (for example, modulation, coding rate, antenna configuration) as well as a different Hybrid Automatic Repeat reQuest (HARQ) entity. On the other hand, in the second case there is a unique HARQ entity for all the CCs. The difference between the alternatives is shown in Figure 3.2. Both schemes have advantages and disadvantages. Minimum changes in the specifications will be required if scheduling, Multiple-Input Multiple-Output (MIMO), link adaptation and HARQ are performed over groups of carriers of 20 MHz. For instance, a user receiving information in 100 MHz bandwidth will need five processing chains for reception in the physical layer, one per each 20 MHz block. Moreover, this alternative is backward-compatible with legacy technologies, as LTE or IEEE 802.16e. On the other hand the main benefit of using only one HARQ process is that the longer the transport block size the more efficient the channel coder is.
Figure 3.2 Two alternatives for the mapping to the components carriers

LTE-A and WiMAX IEEE 802.16m, use Turbo Code (TCs) as a mandatory channel coding. However, the IEEE 802.16m standard proposes Low-Density Parity-Check (LDPC) codes as an additional alternative. Both coding methods are close to the Shannon limit and can achieve low bit error rates for low Signal to Interference plus Noise Ratio (SINR) applications. In general, turbo codes have good performance for all block sizes once a certain minimum length is surpassed. This, together with the high computational complexity of TCs, motivates that packet data units are segmented in several turbo blocks before coding. On the contrary, LDPC codes tend to behave better than TCs with larger blocks, most of all when increasing the SINR. This effect is due to the segmentation of the transport block in several turbo blocks. When the number of turbo blocks increases it is unlikely to complete successfully the transmission of all blocks, hence reducing the system's effective throughput. On the other hand, with a single LDPC block channel coding becomes more robust.
Given that 4G technologies will support bandwidths up to 100 MHz, the number of transmitted bits will increase. This section therefore compares the performance of LDPC codes and TCs with increasing bandwidths or, which is the same, with larger coding blocks. Another open question in case of usage of TCs is the convenience of the segmentation. Precisely, this segmentation has not been carried out in the analysis of this section. The maximum block size that the turbo coder can process is 6144 bits whereas with LDPC this restriction does not exist. Thus, in order to be totally fair in the comparison between these two schemes, segmentation should be taken into consideration.
In the comparison of LDPC codes and TCs, several coding rates and modulations have been simulated. However, for the sake of clarity only two types of simulation assumptions (QPSK with coding rate 1/2 and 16-QAM with coding rate 1/2) are depicted in Figure 3.3. This figure shows a difference of performance lower than 0.3 dB. The parameters of these simulations are listed in Table 3.1. Note that, for a given bandwidth, the guard bands have been accounted for. For instance, for a bandwidth of 100 MHz, 110 Resource Block (RBs) have been simulated instead of 100 RB. Depending on the coding rate and the modulation, this difference is more or less significant. However, this difference of performance has a relative importance due to its value. The most important conclusion is that both LDPC and TC perform very similarly similar for these block sizes.
Figure 3.3 Performance comparison between LDPC codes and TCs for (15156 bits, rate = 1/2) (left) and for (50576 bits, rate = 5/6) (right)
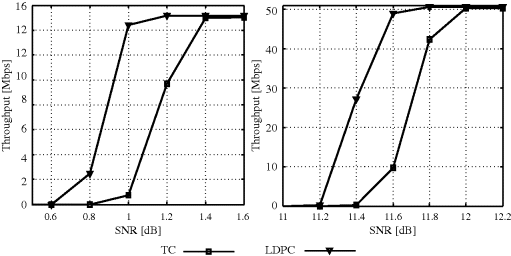
Table 3.1 Basic parameters used in the performance assessment.
Parameter | Value |
Modulation | QPSK/16-QAM |
Channel model | AWGN |
Channel coding | LDPC/TC |
Coding rates | 0.5/0.83 |
Number of RBs | 110 (Figure 3.3) |
Matrix H | WiMAX (IEEEP802.16e 2005) |
Decoding algorithm | Sum-product |
Number of iterations | 100 (LDPC)/8 (TC) |
Segmentation | No (LDPC)/Yes (TC) |
Finally, as shown in Figure 3.4, when the encoded block increases its size there is a clear benefit in not segmenting the transport block. In this case, there is a difference of more than 2.5 dB in the SINR value required to reach the maximum throughput, that is, without any failure in the transmission. The reason is that when the number of turbo blocks increases it is unlikely to successfully complete the transmission of all blocks. Note that this maximum difference between TCs and LDPC codes occurs only in limited operating conditions.
Figure 3.4 Performance comparison between turbo codes with packets no larger than 6144 and LDPC codes for (126476 bits, rate = 5/6) and QPSK

3.3.2 Scheduling
In IMT-Advanced systems two or more CCs belonging to the same of different frequency bands can be aggregated. It is therefore possible to schedule several CCs to a single user. In the assessment of the scheduling methods in CA, the Proportional Fair (PF) scheduler is going to be used due to its simplicity and good performance. This method allocates a RB to the user i* who maximizes its instantaneous data rate over its average data rate (see Equation (4.8)). At slot s, Ri(n, s) is the instantaneous transmission rate on the RB n for the user i and is its average data rate.
PF will be compared with the Round Robin (RoR) scheduler, which allocates full resources to a single user in a circular sequence.
One of the more challenging topics of the resource scheduling in CA is to determine which aggregation strategy is preferable from the system performance point of view. In case of noncontiguous aggregation two scheduling strategies have been investigated in WINNER+ (2009): (1) joint PF scheduling of all RBs available in the aggregated bands and (2) separate and independent PF executed in any aggregated band. For contiguous aggregation, only joint PF scheduling of all available resource blocks has been employed. For the performance evaluation a system-level simulation in the DL of a single-cell scenario has been be considered, without any interference. Channel model corresponds to the Urban Macrocell (UMa) scenario specified in the IMT-Advanced guidelines (ITU-R 2009b). An aggregation of three component carriers of 20 MHz was assumed. A full buffer traffic model was considered. Link adaptation was used, where the modulation scheme was adaptively controlled based on the achieved SINR. In order to evaluate all the considered schemes, a simple PF scheduler was employed. Due to the lack of inter-cell interference, and with the aim of comparing the different aggregation approaches in low SINR regions, the total transmit power of the BS was tuned from −6 up to 10 dBm. The comparison was carried out for 20 users uniformly distributed in the cell. Figure 3.5 present the total cell throughput obtained. The clear advantage of noncontiguous over contiguous aggregation can be noticed, especially when the transmitted power is low and the number of users is high. This is due to the higher spectral diversity experienced when noncontiguous aggregation is performed. However, this comes at the expense of hardware redundancy since more than one physical layer (and possibly MAC) processing chain should be available. Moreover, an interesting feature is that higher cell throughput has been achieved for separate CC scheduling than for joint scheduling of all aggregated bands.
Figure 3.5 Cell throughput achieved with 20 users per cell
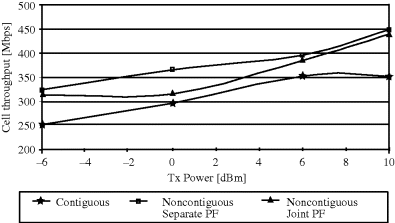
In a similar way to WINNER+ (2009) the QoS performance of two multiuser scheduling schemes called Separated Random User Scheduling (SRUS) and Joint User Scheduling (JUS) are compared in Zhang et al. (2009). At the BS the traffic to the users was buffered in different First Input First Output (FIFO) queues. SRUS method allocates users to only one of the CCs in a random and uniform way and uses a different scheduler for each CC. Evidently, this scheme is not efficient because a CC could be unused while the other is still busy. On the other hand, the JUS method can assign resource of all CCs with a common scheduler. JUS increases the complexity of the scheduler but achieves the maximum spectral efficiency. In a full buffer scenario, the simulation results show that cell throughput of both schemes are similar using (RoR), whereas in the case of PF the gain is between 5–7 between both schemes. Assuming real traffic, the average user latency of SRUS is larger than JUS by around 90–100% when the arrival rate is small. However, as the traffic load increases the performance becomes increasingly similar.
In Chen et al. (2009), the authors made a similar study. In this case, the results show that CA always entails higher throughput than the independent use of CCs. The benefit of dynamic load balancing is also showed. Moreover, the fairness among users is only guaranteed when CA is used. In case of uncoordinated use of CCs this fairness is lost, most of all when users' allocation is not symmetrical.
In Wang et al. (2010b) the authors considered two schedulers based on the PF concept. The first uses Equation (3.1) independently for each CC. As for the second, user throughput is averaged over all aggregated CCs. This last method increases fairness among users. In a full buffer scenario the results show that there is no significant difference between both methods in terms of cell throughput. However, in terms of coverage, the second method has a meaningful gain, 50% when 20% of users can use CA and 90% when this percentage increases up to 50%.
CA and Coverage
Aggregation of lower frequency bands, for example, 450–470 MHz or 698–862 MHz bands, yields better results, as the signal attenuation is lower. It is known that path loss and doppler effect depend on the frequency.
The higher the frequency the higher the path loss is. Consequently, not all the frequency carriers have the same coverage. It will cause the users located at the cell edge to be scheduled on fewer carriers, while others can be scheduled on the entire aggregated spectrum. Fairness could therefore be lost when CA is used.
Figure 3.6 shows an example where two CCs are aggregated. Users near the BS (e.g. UE1) can use both CCs (f1 and f2) whereas users on the cell edge (e.g. UE2) can only use one frequency carrier f1.
Figure 3.6 Coverage of a cell with two CCs

These challenging problems have attracted the attention of the scientist community. For instance, in Songsong et al. (2009) a scheduling method based on user grouping is proposed. Users are divided into groups according to the number of carriers they can be scheduled on. The path loss equations depend on the frequency carrier and the distance between the UE and the considered BS. It is therefore easy to obtain the coverage radius for a certain frequency carrier, assuming that the cell is circular. Hence, each CC will have a maximum radius in which it is possible to operate. After grouping, users are scheduled based on the PF method but taking into account the probability that users fall within the coverage of one or several carrier. In the proposed method, users' throughput is adjusted proportionally to the number of carriers on which they can be scheduled. Simulation results show higher fairness with the proposed method at the expenses of an increasing throughput degradation with the number of users.
So far, all analyzed studies are focused on the DL. However, although there are some similarities in CA between DL and UL, there are also some differences. Due to the limited transmission power of the UE, increasing the bandwidth does not always result in an increase of the user performance. In Wang et al. 2010a an adaptive power control algorithm is proposed in order to compensate these variations in the allocated bandwidth. Firstly, the transmission power on each CC is independently estimated based on the allocated resources and the CC-specific power control parameters. Next, if the total estimated power is higher than the UE maximum power, then the transmission power in each CC is reduced by the same offset. Two types of users were considered in Wang et al. 2010a: Rel-8 users were only allocated to one CC while LTE-A users were assigned to either one CC or two CCs based on their path losses. The simulation results show that the cell edge user throughput of LTE-A and Rel-8 users is the same. However, the average user throughput in LTE-Advanced is higher. The gain achieved with carrier aggregation depends upon the system load, being higher for lower loads.
3.3.3 Channel quality indicator
Orthogonal Frequency Division Multiplexing (OFDM) systems are particularly efficient when link adaptation and user multiplexing in the frequency domain are employed. However, it involves an instantaneous knowledge of the channel quality. Frequency Selective Scheduling (FSS) significantly improves system performance. Depending on the Channel Quality Indicator (CQI) bandwidth used, explicit CQI feedback for every RB can result in significant overhead and, therefore, reduced capacity. In case of reducing excessively the CQI bandwidth the FSS performance benefit could decrease. This is aggravated in LTE-Advanced because multiple CCs can be located in different bands in which the doppler frequency is different. This fact is directly related to the required CQI reporting period.
An efficient and flexible technique for CQI reporting would optimize the tradeoff between the system performance of a FSS algorithm and the uplink bandwidth occupancy. It could therefore be useful to define a flexible CQI reporting method to select a certain level of granularity in the time domain and in the frequency domain depending on the radio channel conditions the UE experiences.
3GPP designed the Rel-8 CQI reporting method as follows:
- The CQI table comprises 16 entries (CQI is 4 bits) (3GPP 2010).
- The table is defined in terms of channel coding rate and modulation scheme.
- An UE reports a CQI index corresponding to a transport format with 10% BLock Error Rate (BLER) target at the first transmission, over the set of RB corresponding to the CQI value.
The eNodeB (eNB) controls time and frequency resources used by the UE. The UE transmits the CQI reports periodically on the Physical Uplink Control CHannel (PUCCH) for subframes without the Physical Uplink Shared CHannel (PUSCH) transmission and on the PUSCH for those subframes with scheduled PUSCH transmissions.
The proposed innovation is based on building a lookup table mapping the doppler frequency fd and the delay spread στ that characterize the channel conditions with the required periodicity of CQI reports in frequency and time, expressed in number of RBs (Nf) and TTI (NTTI) respectively. This lookup table was built using system level simulations WINNER+ (2009). Table 3.2 depicts this lookup table. The gain achieved when using variable reporting periods is assessed in terms of the tradeoff between the system performance and the uplink overhead.
Table 3.2 Look-up table.
Parameter | Value |
![]() |
![]() |
![]() |
![]() |
... | ... |
![]() |
![]() |
Simulations were conducted over a dynamic system-level simulator that follows the indications of ITU-R (2009b) and using the scenarios in Table 3.3. The technology used in the assessment was LTE in its Frequency Division Duplex (FDD) version. Nevertheless, a nonstandard method of CQI reporting was used. The reporting period can be configured as a multiple of 1 ms. Moreover, it was assumed that the UE sends a CQI report with information about the whole bandwidth but with a configurable granularity in frequency. Frequency granularity is expressed in terms of number of LTE RBs. Specifically, the values used in this assessment were 1, 2, 5, 10, 25, 50 RBs for the reporting bandwidth and 1, 2, 4, 8, 16, 32, 64, 128 ms for the reporting period. More details on the simulation assumptions can be found in WINNER+ (2009).
Table 3.3 Simulation scenarios used in the CQI analysis.
From the obtained results it can be concluded that, in all scenarios when the reporting period increases, significant degradation of the spectral efficiency is observed. This degradation is more or less significant depending on the specific scenario. The results are shown in Figure 3.7 where each pair of numbers represents the reporting period and reporting bandwidth respectively. Based on the desired tradeoff (between the maximization of the cell efficiency and the minimization of the uplink overhead) one can identify, in the figure, the optimal pair (number of TTI – number of RBs).
Figure 3.7 Cell spectral efficiency versus uplink overhead for UMa, UMi and RMa scenarios. Each pair of numbers represents the reporting period and reporting bandwidth, respectively
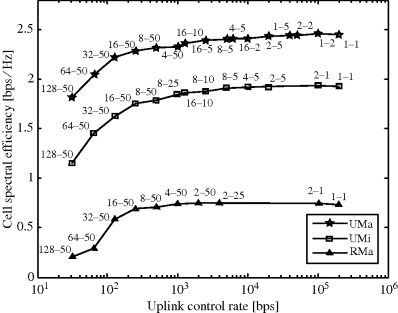
3.3.4 Additional Research Directions
This section aims to sumarize additional research directions that represent open questions to be solved in the future. The research lines focus on fractional soft handover in CA (Chang et al. 2009), flexible carrier aggregation for the home base station (Li et al. 2009) and neighbor carrier signal-strength estimation Yuan et al. 2010).
In Chang et al. (2009) the authors proposed a fractional soft handover scheme. In the LTE standard only hard handover is allowed. With the proposed soft handover, packets are forwarded from the original eNB to the target eNB before breaking the communication. In order to improve the handover scheme, the technique sets one CC as a special carrier for the user to establish a Radio Resource Control (RRC) connection with the target eNB. The authors compare the proposed soft handover method based on CA with the legacy hard handover mechanism and other conventional soft handover procedures. The new proposal reduces the outage probability while saving radio resources and power use.
In Li et al. (2009) the authors studied how to aggregate carriers for Home Base Station (H-BS). They proposed three types of CA allocation patterns: fixed, random and autonomous. In the former, the carrier aggregation is fixed as defined by the operators. In the second case, H-BS selects its CC randomly from the available set of RB when it is turned on or when the channel quality is unbearable. In the latter case, the bandwidth could be divided in components of certain size that H-BS can choose according to interference measurements. The results demonstrate that flexible carrier aggregation mechanisms improve system performance even when H-BS have cochannel interference from the surrounding macrocells. A gain of between 2 to 8 dB is achieved as compared with fix assignment.
Finally, the UEs need to measure periodically the signal strength from all the CCs. Obviously, these measurements entail a rather high cost. In Yuan et al. (2010) the authors proposed to measure the center CC in each band and use correlation to extrapolate results to neighbor bands, thus reducing measurement costs. Evidently, this technique suffers from higher errors when increasing carriers distance. However, for distances around 40 MHz this error is less than 1 dB. On the other hand, in order to reduce the fast-fading impact, the final estimation is filtered, which reduces the estimation error.
3.4 Cognitive Radio Enabling Dynamic/Opportunistic Carrier Aggregation
As earlier stated, CA enables bandwidth resources to be increased and fully utilized in order to provide enhanced peak data rates to service-demanding users. In this context, the CR paradigm Akyildiz et al. (2006); Haykin (2005); Mitola and Maguire (1999), may further extend these benefits by adopting the Dynamic Spectrum Access (DySA) concept (Zhao and Sadler 2007). As a result, Cognitive Component Carrier (CCCs) can be aggregated, in addition to CCs, on an opportunistic and noninterfering basis. In this case, we may appropriately coin the term Opportunistic Carrier Aggregation (OCA) as a method that efficiently uses the spectrum resources by aggregating both CCs and CCCs when available. Accordingly, OCA provides extended capabilities and improved flexibility in the aggregation of spectrum resources, therefore enhancing both data rates and spectrum utilization requirements.
In the following, we will refer to an OFDM-based cognitive radio system, which is able to aggregate carriers in bands belonging to licensed legacy systems (i.e. primary systems), in addition to those bands being licensed to the cognitive (or secondary) system. In this case, the cognitive system may enhance its spectrum access, and consequently its performance, by means of aggregating unused primary spectrum resources. Accordingly, Figure 3.8 represents the case where contiguous and noncontiguous spectrum bands are aggregated within a same band (intraband) or between different bands (interband). Note that “traditional” carrier aggregation is carried out within licensed bands to the secondary system (i.e. spectrum bands A and part of B). On the contrary, opportunistic carrier aggregation is performed within licensed bands to the primary system when these are available – that is, spectrum bands C and part of B.
Figure 3.8 Carrier aggregation and opportunistic carrier aggregation

3.4.1 Spectrum Sharing and Opportunistic Carrier Aggregation
It is widely held that traditional Fixed Spectrum Assignment (FSA), where spectrum is licensed for exclusive use to a licensee, leads to inefficient and poor use of expensive and scarce spectrum resources Akyildiz et al. (2006); Haykin (2005). In this sense, the concept of Dynamic Spectrum Access (DySA) has gained increased momentum in recent years. DySA advocates a more flexible use of the spectrum owned by the licensee (also known as the primary system) through well defined spectrum-sharing mechanisms with a cognitive (or secondary) system (Döttling et al. 2009, Chapter 11).
In this context, the suitability of OFDM-based systems has been widely suggested and recommended given its demonstrated spectral flexibility (Weiss and Jondral 2004). This property enables it to exploit spectrum opportunities fully during primary idle periods by selecting an adequate set of subcarriers for transmission, that is, those falling in detected unused bands, while preventing the use of those subcarriers that fall within detected occupied bands. Hence, OFDM access provides an adaptive transmit filter enabling flexible and opportunistic access to the licensed spectrum.
The use of OFDM-based systems by the secondary system for OCA, in addition to the standard licensed use, comes at a reduced cost because a particular set of subcarriers may be fed by zeroes in the corresponding transmitter Inverse Fast Fourier Transform (IFFT) input to prevent interference with the primary system. At the receiver, the FFT operation implemented to recover the transmitted data will still be valid for the OCA operation mode, thus coming at no extra cost. Implementation issues for DySA using Orthogonal Frequency Division Multiple Access (OFDMA) schemes can be found in Poston and Horne (2005); Rajbanshi et al. (2006) and references therein. Particularly in Poston and Horne (2005), the authors propose the adoption of Discontiguous OFDM (DOFDM) for DySA in idle television channels. They provide a preliminary description of a DOFDM prototype and describe some special considerations for its implementation. Similarly in Rajbanshi et al. (2006), an efficient implementation of a Non-Contiguous OFDMA (NC-OFDMA) transceiver is presented for cognitive radio applications. In this work, due to the deactivation of several carriers during the IFFT procedure at transmission, the authors propose a pruning mechanism for the FFT at the receiver in order to reduce the execution time. Results show improved performance with respect to other pruning methods when medium to large number of subcarriers have been deactivated.
Several DySA implementations arise according to the way in which spectrum is shared between two or more network entities. These implementations may be broadly categorized into three models Zhao and Sadler (2007): the Dynamic Exclusive Use Model, the Open Sharing Model and the Hierarchical Access Model, which are briefly described below (see Figure 3.9).
Figure 3.9 Spectrum access taxonomy

- The Dynamic Exclusive Use model allows a spectrum owner (i.e. the licensee) to grant spectrum access rights over a certain spectrum band to a cognitive system. This cognitive system can make exclusive use of the spectrum as long as it follows the rules provided by the licensee.
- The Open Sharing Model advocates for an open access to shared resources with no exclusive or priority rights over the considered spectrum. In this case, fairness must be guaranteed among users accessing the shared spectrum.
- The Hierarchical Access Model provides an access structure based on primary (or licensed) and secondary (or cognitive) users. In this case, the primary system opens its spectrum so that secondary users may access it in a noninterfering manner.
Based on the above descriptions, OCA will inherit the same DySA paradigms and implementations. In the case of OCA, shared spectrum resources in licensed bands will be identified in order to accommodate so-called CCCs for subsequent CA. This process will not only require the successful detection of available spectrum (i.e. white spaces) but also the determination of the suitability of such bands for CA. In addition, each UE in the considered scenario may, in turn, observe different spectrum availability conditions, thus adding increased complexity to the CCCs selection process regarding whether or not to aggregate a particular CCCs to a particular UE. Furthermore, DySA scenarios are dynamic in nature meaning that available spectrum conditions may suffer from high variability. Then, OCA procedures should be spectrum agile in the sense that they should vacate spectrum suddenly occupied by the licensed system. All these issues call for efficient functionalities to be implemented, such as spectrum awareness, CCCs identification and selection, along with spectrum mobility. These functionalities will be addressed in the subsequent sections.
3.4.2 Spectrum Awareness
Spectrum awareness is a key concept enabling nonintrusive DySA, extensible to nonintrusive OCA, to shared spectrum resources. It essentially provides a set of mechanisms capturing information on the occupancy of a particular spectrum band in a particular geographical area and at a given instant in time.
Several methods and mechanisms providing spectrum awareness information have been identified in the literature, among them: Spectrum Sensing, Geo-Location Databases and Beacon Signaling have attracted major attention. A short overview of these methods is given in the following. For detailed information, the reader is referred to Appendix B.
Spectrum Sensing
Spectrum sensing is the task of obtaining awareness about the spectrum usage and existence of primary users in a particular geographical area. This awareness is usually undertaken locally by the secondary user, which performs measurements over a particular radio frequency band. While spectrum sensing usually refers to the energy measurement over a given spectrum band, it actually encompasses broader connotations on obtaining spectrum usage characteristics across multiple dimensions such as time, space, frequency and code (Yucek and Arslan 2009). It also involves determining what types of signals are occupying the spectrum including the modulation, waveform, bandwidth, carrier frequency, etc.
Geo-Location Databases
Spectrum awareness by means of geo-location databases operates as follows. An unlicensed (secondary) device estimates its position and checks a database in order to identify those spectrum bands that are being used by licensed (primary) services in the vicinity of the unlicensed device. This implies that the device must have an estimate of its position, an estimate of its position error, an estimate of its interference range, and access to a database of potential licensed service areas (Brown (2005)).
Beacon Signaling
In the context of spectrum awareness, beacon signals can be utilized to indicate if a particular licensed spectrum band is occupied, and thus available for secondary access (Hulbert 2005). Beacon signaling would ease the performance requirements of spectrum sensing by secondary devices and also facilitate the delivery of database information in absence of Internet connectivity. Beacons may advertise either permission (grants) for cognitive radios to access spectrum, or alternatively, denials of spectrum access for cognitive radios, see, for example, CEPT-ECC (2010); Hulbert (2005). However, as shown in Mangold et al. (2006), a dual beaconing approach may offer greater reliability than using a single beacon either for grant or for denial. Furthermore, beacon broadcasting can be used within a network to inform neighbors about available communication channels (Zhao et al. 2005).
3.4.3 Cognitive Component Carrier Identification, Selection and Mobility
The selection of bandwidth-limited CCCs may impose high constraints in terms of signaling and increased complexity while not providing substantial aggregated spectrum and thus limiting increased throughput (see for example, in Figure 3.8, the potential CCCs identified in spectrum band C for UE2). Moreover, particular primary bands may present high variability in terms of usage, meaning that potential CCCs lying in these bands must be frequently vacated and reassigned, which, again, increases the burden of signaling. For the same reasons, the cognitive system will preferably aggregate intraband contiguous spectrum (refer to Figure 3.8 case (d)) rather than intraband noncontiguous (Figure 3.8 case (e)) or interband noncontiguous (Figure 3.8 case (f)) aggregations.
Upon service demand by a particular UE, radio and spectrum resource management mechanisms will provide efficient algorithms for the assignment of CCs and CCCs to the existing users. In this sense, we may identify carrier selection policies that operate upon session establishment (intersession carrier selection) and during the session's duration (intrasession carrier selection). For the case of intrasession carrier selection, existing mobility mechanisms of traditional networks should be extended in order to support spectrum-agile intraband and interband handoffs between CCs and CCCs in both contiguous and noncontiguous bands. Different UEs may be configured with a set of different CCs and/or CCCs, according to their hardware capabilities, channel conditions, QoS requirements and spectrum availability among other issues. In this way, OCA adds a new dimension, that is, spectrum availability, into the already complex process of CA.
3.5 Implications for Signaling and Architecture
When using carrier aggregation, some signaling information related to the allocation of the resources is provided. As previously mentioned, the 3GPP is currently addressing this specific topic where backward compatibility and the reduction of complexity are being considered. However, with the objective of having maximum system performance, all cases must be studied.
There are five key aspects that must be established with respect to signaling in CA:
1. CC discovery and accessibility. Among the configured CCs, at least one downlink CC should transmit the required broadcast information to enable UEs to access the system. In order to increase flexibility and guarantee backward compatibility, the most reasonable proposal is that all CCs are accessible to all UEs, being Release 8 or 10. Those UEs with the capability to support multiple CCs will detect the stronger CC in the initial access and receive system information from all CCs, as this system information will be common among aggregated CCs. However, if any CC system information exists, this should be transmitted by each CC in a different signaling channel. It must be noted that, for the sake of simplicity, the synchronization of all of the aggregated carriers is required, with respect to both time and frequency.
2. Once synchronized, the UE will use a random access channel to connect to the system. If the CA is symmetric between UL and DL then the DL CC will coincide with its paired UL carrier. However, in the asymmetric carrier aggregation case, in which multiple DL CCs may be associated with only one UL CC, the configuration between UL CC and DL CC may cause a serious ambiguity problem as the BS may not know which DL CC is selected by the UE. In order to solve this problem CCs must be identified, transmitting this information in the corresponding signaling broadcast channel. Besides, this identifier simplifies the scheduling process and allows future handovers between CCs.
3. Another important aspect is related to the downlink signaling control for the scheduling. In LTE-A UEs must be able to decode control channel DL information on multiple DL CCs. Besides, any control channel on a CC can assign resource blocks in both DL and UL in one of multiple CCs. Therefore, the CC identifier is needed to specify to the UE on which component carrier the control channel assigns resources. The BS could decide on transmitting the scheduling information of the whole aggregated band several times, one per CC, or could limit this transmission to just the best CC. In IEEE 802.16m the situation is simpler, since each user has only one primary carrier with all control information. However, data can be transmitted in the primary plus in one or several secondary carriers. Again CC identifiers are needed to identify the exact allocation of resources.
4. In LTE-A and additional control channel reports on the HARQ status. Again, in case of asymmetry it will be required to select the DL CC on which this channel is transmitted. The most reasonable alternative is to select only the best CC, although this issue requires further research. In IEEE 802.16m all control information is transmitted through the primary CC and therefore this problem does not exist.
5. In the uplink, in case of a multiple CC assignment, the UE may have multiple HARQ processes in parallel, one per CC. This would mean that multiple ACK/NACK messages corresponding to the DL CC transport blocks should be transmitted using the UL control channel. Moreover, the UE will also feedback multiple channel state indicators, including optimum MIMO precoding matrices, one per CC. This is the information used for CC handover and hence it is important to guarantee its proper reception. Again one UL control channel could report about all the UL CCs using the CC identifiers. The decision on transmitting several parallel UL control channels will lie again on the BS design.
With regards to the channel state reports, it has been confirmed in WINNER+ (2009) that significant overhead reduction can be achieved if the carrier and scenario are known. Flexibility in the reporting mechanism is therefore recommended. The Channel State Information (CSI) reporting procedure can be performed automatically by the UE depending on the frequency carrier that UE is using to communicate with the base station and depending on the speed that characterizes its radio channel. In this case no specific requirement on signaling and specific measurement would be expected. Nevertheless, assuming that the BS is able to know the lookup table used by the UE and/or assuming that the BS can set its own lookup table in the UE, the BS could decide to force the UE to change the granularity of the CSI reporting referring to another pair (number of frequencies, number of time slots) contained in the lookup table in order to optimize its radio resources allocation strategy. In such a scenario, a new signal is expected from the UE to the BS where the UE communicates the lookup table to the base station every time that the UE is going to camp on a new cell or a new signal is expected from the BS to the UE where the BS communicates its own lookup table every time that a new UE is going to be served. %Moreover, in this scenario it is expected a new signalling from the BS to the UE where the BS communicates the UE to change the level of CSI reporting granularity to be used every time that the BS wants to force the UE to use a new combination (number of frequencies, number of time slots) of the look-up table.
3.6 Hardware and Legal Limitations
Due to hardware limitations at the UE, in a short time it is expected that no more than 8192 FFTs (213) will be implemented by vendors. Considering LTE subcarrier spacing, this results in no more than 120 MHz. For IEEE 802.16m with component carriers of 10 MHz, the maximum bandwidth is reduced to 80 MHz. Lower bandwidth can be configured by transmitting zeros in the unused subcarriers. Another approach to aggregate spectrum is not to increase FFT size but to use multiple RF transceivers. In this case, although the guard bands of each component carrier cannot be utilized, the hardware complexity is lower and backward compatibility is easily reached.
Another issue is the existence of such a large continuous bandwidth. In fact, the result of WRC 2007 may not allow such wide carrier bandwidth for new radio systems. Given the current spectrum distribution, the only valid alternative to process the entire frequency band from about 400 MHz to about 6 GHz seems to be the use of parallel receivers for each different band.
This may lead to another hardware limitation, due to antennas, at least on the mobile side. Indeed, the antenna should cover the whole set of bands decided by WRC 07, especially the lower band (698–960 MHz) (not to mention 400 MHz). If we assume its size should remain compatible with that of a smartphone, then its electrical dimension (dimension over wavelength) in the 698–960 MHz band will be about 0.25, and its relative bandwidth (bandwidth over central frequency) should be about 0.3. For these values, electromagnetic theory predicts a maximum possible radiation efficiency (ratio of power actually radiated to the power put into the antenna terminals) of about 0.25, which is quite poor. Indeed, radiation efficiency decreases as the antenna length decreases and for larger relative bandwidths. Therefore, alternative solutions should be found, such as frequency reconfigurable antennas.
The last hardware limitation is the difficulty for the RF part when aggregation is performed on noncontiguous bands. As explained in (Shukla et al. 2006) there is a research challenge related to the mitigation of intermodulation distortion, especially if fragments share a transmit/receiver chain, or chains need combining to share an antenna or amplifier, to reduce component count and overall size.
3GPP 2010 Physical layer procedures. Technical Specification Group Radio Access Network 36.212 v9.2.0, 3rd Generation Partnership Project (3GPP), http://www.3gpp.org/ftp/Specs/html-info/.
Akyildiz I, Lee W, Vuran M and Mohanty S 2006 Next generation/dynamic spectrum access/cognitive radio wireless networks: A survey. Computer Networks 50 (13), 2127–2159.
Brown T 2005 An analysis of unlicensed device operation in licensed broadcast service bands Proc. First IEEE International Symposium on New Frontiers in Dynamic Spectrum Access Networks, pp. 11– 29.
CEPT-ECC 2010 Technical and Operational Requirements for the Possible Operation of Cognitive Radio Systems in the “White Spaces” of the Frequency Band 470–790 Mhz (Annex 3 to Doc. SE43(10)103). Technical report, Conférence Européenne des Post et Telecommunications, http://www.ict-qosmos.eu/project/standardisation-and-regulation/ceptecc-se43.
Chang J, Li Y Feng S, Wang H, Sun C and Zhang P 2009 A fractional soft handover scheme for 3GPP LTE-advanced system Proc. ICC 2009 – IEEE Int. Conf. Commun., pp. 1– 5.
Chen L, Chen W, Zhang X and Yang D 2009 Analysis and simulation for spectrum aggregation in LTE-Advanced system Proc. VTC 2009 Fall – IEEE 70th Vehicular Technology Conf., pp. 1– 6.
Döttling M, Mohr W and Osseiran A 2009 Radio Technologies and Concepts for IMT-Advanced. John Wiley & Sons, Ltd., Chichester.
Haykin S 2005 Cognitive radio: brain-empowered wireless communications. IEEE Journal on Selected Areas in Communications 23 (2), 201–220.
Hulbert A 2005 Spectrum Sharing Through Beacons Proc. PIMRC 2005 – IEEE 16th Int. Symp. on Pers., Indoor and Mobile Radio Commun., pp. 989– 993, Berlin, Germany.
IEEE 802.16 2010 System Description Document (SDD). Document IEEE 802.16m-09/0034r3, IEEE 802.16 Broadband Wireless Access Working Group, Task Group m, http://www.ieee802.org/16/tgm/core.html.
IEEE 802.16e 2005 Draft IEEE Standard for Local and Metropolitan Area Networks. Part 16: Air Interface for Fixed and Mobile Broadband Wireless Access Systems. Amendment for physical and Medium Access Control Layers for Combined Fixed and Mobile Operation in Licensed Bands. Document IEEE P802.16e/D12, Technical Specification Group Services and System Aspects, http://ieeexplore.ieee.org/xpl/mostRecentIssue.jsp?punumber=4039740
ITU-R 2008a Requirements Evaluation Criteria and Submission Templates for the Development of IMT-Advanced. Report ITU-R M.2133, International Telecommunications Union Radio (ITU-R), http://www.itu.int/publ/R-REP/en.
ITU-R 2008b Requirements related to Technical Performance for IMT-Advanced Radio Interface(s). Report ITU-R M.2134, International Telecommunications Union Radio (ITU-R), http://www.itu.int/publ/R-REP/en.
ITU-R 2009a Definitions of Software Defined Radio (SDR) and Cognitive Radio System (CRS). Report ITU-R SM.2152, International Telecommunications Union Radio (ITU-R), http://www.itu.int/publ/R-REP-SM.2152/en.
ITU-R 2009b Guidelines for Evaluation of Radio Interface Technologies for IMT-Advanced. Report ITU-R M.2135-1, International Telecommunications Union Radio (ITU-R), http://www.itu.int/publ/R-REP/en.
Iwamura M, Etemad K, Fong MH, Nory R and Love R 2010 Carrier aggregation framework in 3GPP LTE-Advanced. IEEE Commun. Mag. 48 (8), 60–67.
Li J, Liu Y, Duan J and Liang X 2009 Flexible carrier aggregation for home base station in IMT-Advanced System Proc. 5th International Conference on Wireless Communications, Networking and Mobile Computing, pp. 1– 4.
Mangold S, Jarosch A and Monney C 2006 Operator assisted cognitive radio and dynamic spectrum assignment with dual beacons – detailed evaluation Proc. IEEE 1st International Conference on Communication Systems Software & Middleware, pp. 1– 6, New Delhi, India.
Mitola J and Maguire G 1999 Cognitive radio: making software radios more personal. IEEE Personal Communications 6 (4), 13–18.
Poston J and Horne W 2005 Discontiguous OFDM considerations for dynamic spectrum access in idle TV channels Proc. First IEEE International Symposium on New Frontiers in Dynamic Spectrum Access Networks, pp. 607– 610.
Rajbanshi R, Wyglinski AM and Minden GJ 2006 An efficient implementation of NC-OFDM transceivers for cognitive radios Proc. 1st International Conference on Cognitive Radio Oriented Wireless Networks and Communications, pp. 1– 5.
Shukla A, Willamson B, Burns J, Burbidge E, Taylor A and Robinson D 2006 A Study for the Provision of Aggregation of Frequency to Provide Wider Bandwidth Services. Technical Report R/06/01773, QINETIQ, http://www.aegis-systems.co.uk/download/1722/aggregation.pdf.
Songsong S, Chunyan F and Caili G 2009 A resource scheduling algorithm based on user grouping for LTE-Advanced system with carrier aggregation Proc. International Symposium on Computer Network and Multimedia Technology, pp. 1– 4.
Takagi H and Walke BH 2008 Spectrum Requirement Planning in Wireless Communications first edn. John Wiley & Sons, Ltd., Chichester.
Wang H, Rosa C and Pedersen K 2010a Performance of uplink carrier aggregation in lte-advanced systems Proc. VTC 2010 Fall – IEEE 72st Vehicular Technology Conf., pp. 1– 5.
Wang Y, Pedersen K, S andrensen T and Mogensen P 2010b Carrier load balancing and packet scheduling for multi-carrier systems. IEEE Trans. Wireless Commun. 9 (5), 1780–1789.
Weiss T and Jondral F 2004 Spectrum pooling: an innovative strategy for the enhancement of spectrum efficiency. IEEE Communications Magazine 42 (3), 8–14.
WINNER+ 2009 Celtic Project CP5-026 Intermediate Report on System Aspect of Advanced RRM (ed. Monserrat J. and Sroka P.). Public Deliverable D1.5, Wireless World Initiative New Radio – WINNER+, April 2009, http://projects.celtic-initiative.org/winner+/index.html (accessed June 2011).
WINNER+ 2010 Celtic Project CP5-026 Strategies and technologies for spectrum utilisation and sharing aspects of IMT (ed. Siebert M.). Public Deliverable D3.3, Wireless World Initiative New Radio – WINNER+, http://projects.celtic-initiative.org/winner+/index.html
Yuan P, Xiao D, Han J and Jing X 2010 Neighbor carrier signal strength estimation for carrier aggregation in LTE-A Proc. WASE International Conference on Information Engineering, vol. 1, pp. 284– 287.
Yucek T and Arslan H 2009 A survey of spectrum sensing algorithms for cognitive radio applications. IEEE Communications Surveys and Tutorials 11 (1), 116–130.
Zhang L, Wang YY, Huang L, Wang HL and Wang WB 2009 QoS performance analysis on carrier aggregation based LTE-A systems. Proc. IET International Communication Conference on Wireless Mobile and Computing, pp. 253– 256.
Zhao J, Zheng H and Yang GH 2005 Distributed coordination in dynamic spectrum allocation networks Proc. First IEEE International Symposium on New Frontiers in Dynamic Spectrum Access Networks, pp. 259– 268.
Zhao Q and Sadler B 2007 A Survey of Dynamic Spectrum Access. IEEE Signal Processing Magazine 24 (3), 79– 89.