Chapter 7
Hybrid Electric Vehicles
Conventional powertrain systems with a single power source based on fossil fuels have dominated vehicle design for the past decade. In recent years, however, fuel economy and pollution concerns have caused hybrid powertrain systems to be seen as one of the alternative approaches to powertrain design. Commercial interest in hybrid vehicle technology has grown at a much more dramatic rate than was predicted a decade ago. Around that time, many industry observers were substantially more optimistic about a major leap from current petroleum-based technology straight to hydrogen, fuel cells and biofuel systems. However, it is now widely accepted that hybrid vehicles will have a significant role to play over the next couple of decades as these other technologies continue to be developed.
The growth in interest in hybrid technology has been driven by increasing concerns about the environment and fuel efficiency savings. Petrol emissions in a car can release harmful gases into the air that can contribute to the ‘greenhouse effect’, damaging the environment's protective ozone layer and causing global warming. In addition, petrol is a limited natural resource with potential supply problems in future. Although hybrid vehicles are still reliant on petrol, they offer the flexibility to enhance the fuel economy and emissions of vehicles without sacrificing vehicle performance factors such as safety, reliability and other conventional vehicle features. This has prompted researchers to develop innovative hybrid powertrain configurations and generate design guidelines on component sizing and control strategies.
Hybrid electric vehicles attempt to combine the benefits of petrol engines and electric motors – the engine provides most of the vehicle's power, and the electric motor provides additional power when needed for accelerating and overtaking. This allows a smaller, more efficient internal combustion engine to be used and to be operated near its optimal efficiency range. The electric power for the electric motor is generated not only from the engine but also from regenerative braking that converts otherwise wasted energy from braking into electricity and stores it in the battery.
This chapter is aimed at providing an overall understanding of the main issues of hybrid drivetrains with special emphasis on the power flow and power management.
7.2 Types of Hybrid Electric Vehicles
A hybrid electric vehicle (HEV) is a vehicle fitted with an internal combustion engine and an electric motor. Hybrid vehicles can be designed in different types, a variety of technologies ranging from those using the internal combustion engine as major driving power and those using the electric motor as the main driving source (see Table 7.1). This makes it difficult to group hybrid vehicles into one category and it is possible that more diversified hybrid vehicles will be developed in the future, as hybrid technologies mature.
Table 7.1 Range of hybrid vehicles.
7.2.1 Basic Classification
Hybrid vehicles are traditionally divided into two basic types, namely series and parallel, based on the way the two power sources are utilized in the vehicle. In the series type, the vehicle is mechanically driven by an electric motor and the internal combustion engine is used to produce electric energy. In the parallel type, the mechanical power is delivered to the wheels by either the IC engine or electric motor alone or by both together.
7.2.1.1 The Series Hybrid
In a series hybrid vehicle, an electric motor is responsible for propelling the vehicle. The necessary electric energy is basically produced by a generator that is mechanically driven by an IC engine. Thus, in this type of hybrid system, the main energy source is still the fossil fuel that is used by the IC engine and is transformed into the electric energy that is either stored in the battery pack or directly drives the electric motor that propels the vehicle. This means that in this type of hybrid vehicle the installed IC engine does not drive the vehicle directly and mechanically.
Figure 7.1 illustrates a typical series hybrid configuration in which the main components can be distinguished. The mechanical power from the engine (ICE) drives a generator (G) and the resulting electric current is rectified, and through an electronic converter it is used to either charge the battery pack (B) or drive an electric motor (MG) that can also work as a generator. The motor is controlled by a power electronic device called a motor controller (MC). The mechanical power produced by the motor usually drives the vehicle through a torque amplification device (TAD) attached to the wheels (e.g. a transmission, reduction gear or final drive).
Figure 7.1 A typical series hybrid configuration

It can be argued that the conversion of the IC engine's mechanical power to electrical power and then back to mechanical power will result in more power losses compared with a conventional vehicle with similar engine. This is in general true, however, there are two reasons that might make the series hybrid more efficient than a conventional vehicle. One is the regenerative power obtained from the vehicle kinetic or potential energy (e.g. braking or gravitational forces). The other benefit of the series hybrid vehicles is the independence of the engine operation on the instantaneous vehicle load and speed which allows the engine to work under its most efficient conditions. However, the drawback of the series hybrid vehicle is its large electric motor that is the only source of traction and must generate the total motive force for the vehicle.
The regenerative power is obtained from the electrical power generated by the MG when it is driven by the wheels during braking or downhill travel. The MG acts as a generator, and the electric current (dashed line in Figure 7.1) is absorbed by the battery pack if it is not already fully charged. This two-way mechanical energy flow is shown by the double-ended arrows of Figure 7.1.
7.2.1.2 The Parallel Hybrid
The parallel hybrid type with the example configuration shown in Figure 7.2 uses two different mechanical power flows to propel the vehicle. The mechanical power of either of the two sources can drive the wheels individually or the two sources can work simultaneously and in parallel. The connection of the two mechanical powers is performed in a distributing device MPD that controls the split of power in different ways. The mechanical power from the engine can be fully supplied to the driving wheels or at the same time to the MG (in generator mode). The mechanical output of the MG (in motor mode) alone, can be sent to the wheels or it can be added to the output of the ICE. In regenerative mode, the mechanical power from the wheels drives the MG (in generator mode) and the electrical energy supplied to the battery. In other words, in a parallel hybrid system the mechanical system must incorporate a power-combining device and a regeneration scheme so that the vehicle can be propelled by any combination of the two sources and so that the battery can be recharged either by the engine or by the kinetic energy of the vehicle.
Figure 7.2 A typical parallel hybrid configuration
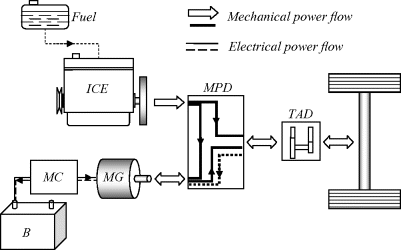
One benefit of the parallel hybrid layout is the lower energy conversion losses since, unlike the series configuration, engine power is directly transferred to the driving wheels. However, the direct connection of the engine to the wheels can also be regarded as a disadvantage due to the associated transient engine operation in inefficient working conditions. In addition, the direct connection of engine to the driving wheels requires a transmission that was absent in the series case. In terms of electric components, in this case, only one MG is used whereas in series configuration there was an additional generator.
7.2.2 Basic Modes of Operation
Both series and parallel hybrid electric vehicles provide several modes of operation depending on the availability of the power sources. The central philosophy in using different modes of operation is to keep the energy usage as low as possible. To this end, therefore, the goal would be the optimal use of the three sources of energy (fossil fuel, battery and vehicle mechanical energy). Typically when the vehicle is started, the ICE is off and the electric energy acts as driving source. The ICE takes over to power the vehicle at cruising speeds and during heavy accelerating or when additional power is needed, the electric energy is also used to propel the vehicle. When the vehicle is stopped for more than a certain period, the ICE is shut off automatically so that energy is not wasted in idling. In these cases the battery continues to power the accessory systems, such as the air conditioning and in-vehicle electrics.
The basic modes of operation can be listed as follows:
1. Pure ICE (Engine-only) mode In this mode, the ICE produces the full power for vehicle motion and no power is used from the batteries.
2. Pure electric (Electric-only) mode In this mode, the ICE produces no power (turned off) and the full power for vehicle motion is taken from the batteries.
3. Hybrid mode The power for vehicle motion in this mode is taken simultaneously from both ICE and batteries.
4. ICE plus charging mode In this mode of operation, the ICE not only produces the power for vehicle motion, but also to recharge the battery.
5. Regeneration mode The kinetic energy of vehicle during braking (or its potential energy during downhill motion) can be used to turn the electric motors and produce electricity. Using energy from the wheels to turn the motor slows the vehicle down. This energy is produced when the battery can accept it and is stored for later use in electric or hybrid mode. In this mode, the ICE is off and the battery is charged by the MG in its generator mode.
6. Charging mode There are cases when the vehicle is at a standstill and no power is used for its motion. In these circumstances if the battery needs recharging, then the ICE is turned on to power the generator and charge the battery.
7. Hybrid charging mode In this case the battery needs more recharging energy than is available through regeneration and the ICE generator units will also contribute to producing electricity during braking.
Table 7.2 summarizes the modes of operation for both series and parallel hybrid types and the components that participate in each mode.
Table 7.2 Component participation in different operation modes.
7.2.3 Other Derivatives
The parallel configuration provides more flexibility in allowing various power flow schemes between the two mechanical power generators. On the other hand, the series configuration also offers some benefits, as discussed earlier, thus combining the two layouts potentially creates even more design opportunities for hybridization.
Many types of hybrid designs have been developed which incorporate the basic concepts discussed earlier, combined with distinct concepts for motive force and power management. No agreed classification for the hybrid designs is available and in most cases a variety of commercial terms is also used. Some types are identified by their technical layout and some by the way in which they use the electric energy. The former types will be considered in the current section and the latter concepts will be discussed in a later section.
7.2.3.1 Series-Parallel Hybrids
A series-parallel hybrid (SPH) configuration is the most common layout in which the benefits of both series and parallel layouts are integrated into a combined system. In an SPH the vehicle must be able to run in both series and parallel modes. In series mode, the traction is produced by the electric motor and the engine power is only used to recharge the battery. For this reason, a generator must be included in the design.
In parallel modes, the IC engine and electric motor can either work independently or together. In addition, the IC engine can contribute tractive power as well as recharging the battery. This combination takes advantage of the efficiency range of the electric motor in the vehicle's low speed range, and the IC engine works in the higher speed ranges of vehicle motion.
Various layouts can be found for the SPH configuration depending on the mechanical power distribution (MPD) system involved. The mechanical power distributor can be used to combine the mechanical power of the ICE and MG as well as splitting the power for generator use as shown in Figure 7.3. Alternatively, the MPD can be used between the ICE and generator, while connecting the motor directly to the driveline. The torque amplification device (TAD) such as a transmission or reduction gear can be placed either before or after the MPD.
Figure 7.3 A typical series-parallel hybrid configuration

7.2.3.2 Power Split Hybrids
The series-parallel types of HEVs are also sometimes called power split hybrid systems, owing to the special transmissions used to combine the mechanical power from two power sources. Power split designs usually use two motor/generator (MG) units in their configurations in order to exercise sufficient control of both speeds and torques in the system. A combination of two MGs allows the engine to drive one of them as a generator to either charge the battery or supply power to the other motor.
Of the numerous power split transmission designs, two configurations known as single mode and dual mode power split transmissions have been commercially successful. The single mode term refers to the fact that there is only one way in which the power is split between the mechanical and electrical paths. A dual mode arrangement has more than one epicyclic gear unit and introduces clutches into the drivetrain which can be used to change the power flow through the system.
The Toyota Hybrid System (THS) is used in cars such as the Prius and Camry hybrid models and its power split device (PSD) enables the engine to operate at its efficient regions, independent of the vehicle speed and in fact it provides an electronically controlled transmission with continuously variable gearing. The power split device, which is a planetary (or epicyclic) gear unit (see Section 7.3), controls the ratio of power going directly to the wheels and to the generator in a continuously variable way (see Figure 7.4). Consequently, there are still energy conversion losses due to the transmission efficiency.
Figure 7.4 Toyota Prius, THS arrangement
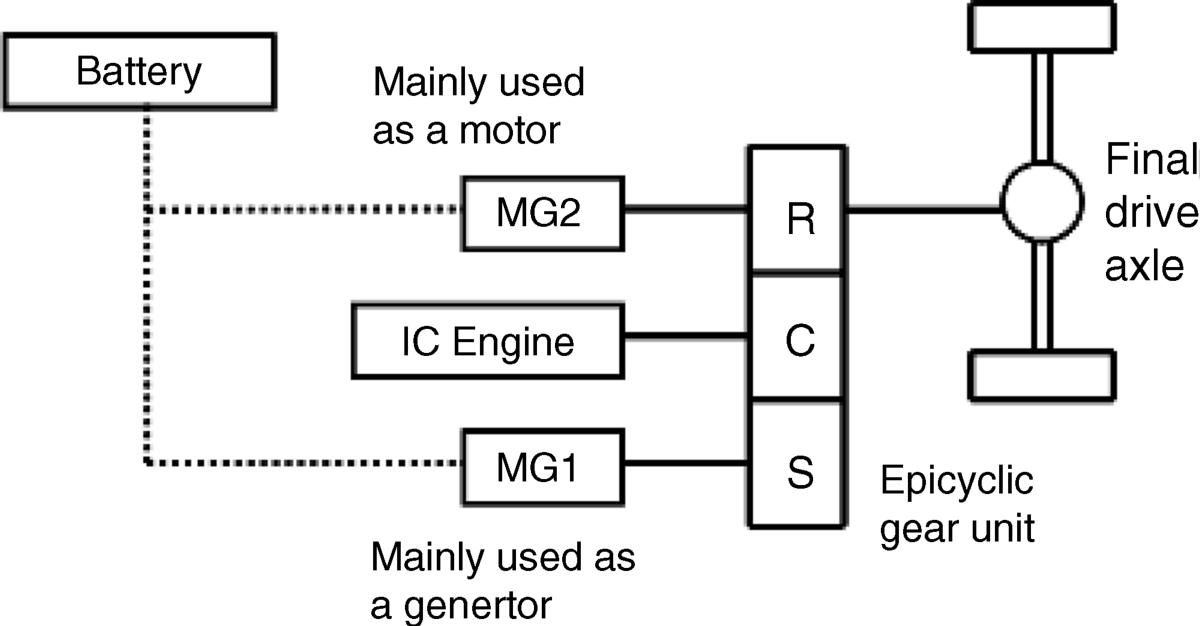
The FHS system, developed by Ford, Volvo and Aisin (Figure 7.5), is very similar to the THS except that one of the MG units is connected to the epicyclic planetary gear via an additional set of ‘output gears’. An alternative version of the single mode design by NexxtDrive (Figure 7.6), employs two epicyclic gears in conjunction with two MG units. This arrangement offers potential efficiency benefits over the single planetary gear systems because there is more flexibility in controlling the balance of power flows through the mechanical and electrical branches. It has an important benefit of providing two points at which the electrical path transmits zero power, and therefore incurs no losses [1].
Figure 7.5 Ford, Volvo, Aisin, FHS arrangement

Figure 7.6 NexxtDrive arrangement

The GM Allison design (AHS) is based on a dual mode arrangement (Figure 7.7) that uses two epicyclic gear units and two clutches. One of the modes remains exactly the same as for the single mode system but the other – the compound split mode – effectively splits the input power into two paths and then recombines them at the second epicyclic unit. A GM/Daimler Chrysler/BMW consortium has released a dual mode system with the arrangement shown in Figure 7.8, offering four fixed gear ratios. It incorporates three epicyclic gear units, linked together via clutches, and although it appears rather complex at first sight, it nevertheless only involves the mechanical content and overall size associated with a modern, multispeed automatic transmission.
Figure 7.7 GM Allison AHS arrangement

Figure 7.8 GM/Daimler Chrysler/BMW power split arrangement

7.2.3.3 Plug-In Hybrids
Electric vehicles (EVs), that use a battery as the only source of energy, must plug into the electric grid to recharge their batteries. HEVs rely on the energy from fossil fuels and their batteries are charged by a generator driven by the ICE. A hybrid vehicle that can also receive electric power from the grid to charge its batteries is called a ‘plug-in hybrid electric vehicle’ or PHEV in short. The size of the battery defines the vehicle's range that can be achieved in electric mode. Therefore, PHEVs need larger battery packs when compared to other HEVs in order to increase their battery working range. In addition to increasing the battery capacity, modification to the vehicle controller and energy management system is also necessary. Additionally, owing to lower electricity costs during night time, recharging the battery at night will be advantageous not only to the vehicle owners, but also to the electricity providers.
7.2.4 Degree of Hybridization
In an electric hybrid vehicle with two different power sources, the balance between the sources can be quantified by a simple criterion defined as the degree of hybridization (DOH):
(7.1)
in which PE and PT are the electric and total installed powers. The electric installed power refers to the maximum power of the electric motor(s) and the total power is the sum of the maximum engine power (PICE) and motor power. Typical variations of the DOH for different engine and motor powers are illustrated in Figure 7.9. Different definitions may also be found in the literature such as the ratio of motor power to ICE power (PE/PICE) which results in higher DOH values.
Figure 7.9 The variation of the DOH with electric to engine power ratio

A different way of classifying HEVs is simply to refer to those utilizing the highest DOH values as full hybrids and those with small values of DOH as light (or micro) hybrids. HEVs with average DOH values are categorized as mild hybrids. In this type of classification, the architectures of hybrid vehicles are not important and so two hybrid vehicles with similar layouts may fall into different categories according to their DOH values. Nonetheless, no specific figures have been agreed for the three hybrid categories and designation of a vehicle into them remains a matter of choice.
7.2.4.1 Full Hybrids
A large degree of hybridization in a full hybrid electric vehicle means that a large electric motor must be used. But this is not the only requirement since a full hybrid needs to allow ‘electric-only’ driving which means the battery must also be large enough for this purpose. In addition, the full hybrid designs provide a full range of hybrid operations including engine start-stop, regenerative braking and motor assist motion.
7.2.4.2 Mild Hybrids
HEV designs with a lower degree of hybridization, even with a similar architecture to that of full parallel hybrids, are often regarded as mild hybrids. In a mild hybrid, only the engine is responsible for moving the vehicle, however, at high loads the motor(s) can assist the engine in producing the motive force. Therefore, mild HEV designs provide engine start/stop, electric assist driving and even regenerative braking, but do not allow driving with the electric motor only.
7.2.4.3 Light/Micro Hybrids
A light hybrid is an HEV with an oversized starter motor that can also be used as a generator, usually called an integrated starter-generator (ISG) or a belted alternator starter (BAS). The ISG must be capable of acting as a motor to crank the engine and drive the belt-driven accessories when the engine is off. Recharging the battery can also be done by the ISG at low engine loads.
Light hybrids are capable of stop/start and regenerative charging functions. Shutting the engine off whenever the vehicle is not moving improves the fuel economy by a few percent. The implementation of a mild hybrid on a vehicle requires only small modifications including a larger electric motor generator and an oversized battery together with simple power electronics.
The term ‘micro hybrid’ is used to differentiate some vehicles in the mild family. In fact, a micro hybrid vehicle is the simplest kind of HEV with very low DOH. The most common definition for a micro hybrid vehicle is a ‘start-stop system’ in which the IC engine is turned off when the vehicle pulls to a stop, and is restarted when the driver accelerates. Like mild hybrids, ISG systems are used and thus similar functions are available. Some micro hybrids, in addition to the start-stop function, use some degree of regenerative braking to charge the battery.
Figure 7.10 compares the four types of HEVs in terms of their functionalities. It should be noted that in general, hybrid vehicles lie in a spectrum between micro and full hybrids and it is sometimes hard to differentiate between the two neighbouring types.
Figure 7.10 Comparison of basic functions among different hybrid types

The transmission design has been a crucial issue in the success of hybrid vehicles. Specific types of transmissions, also often referred to as power split devices (PSD), are of particular importance. A PSD is a mechanical device that makes it possible to control the power share between the ICE and electric motor(s) in a hybrid system. In Section 7.2.3.2 it was briefly explained how the power split hybrids are categorized (see Figures 7.4 to 7.8). In this section, the functioning of a PSD will be discussed in more detail.
7.3.1 Simple PSD
The simplest PSD mechanism is the planetary gear system (or epicyclic system) which has three input/output shafts (see Section 4.4.2). This mechanism, that is widely used in conventional automatic transmissions, is used by Toyota as a power split device in their THS hybrid systems (see Figure 7.4). Figure 7.11 illustrates a typical epicyclic gear set in a schematic form as well as a simplified ‘stick diagram’.
Figure 7.11 A typical planetary gear set (a) schematic and (b) stick diagram

In the THS system, the engine output shaft is directly connected to the carrier C, motor/generator number 1 (MG1) is connected to the sun S and motor/generator number 2 (MG2) is connected to the Ring R. Denoting the angular speeds of engine, MG1 and MG2 as ,
and
respectively, the kinematic relationship among the three angular speeds is (Equation 4.90):
According to the free body diagrams of gears in an epicyclic set (Figure 7.12), the quasi-static relations among the torques applied at input/outputs are (the dynamic and frictional effects are ignored):
Figure 7.12 Free body diagrams of epicyclic gear components

The minus signs in Equations (7.4) and (7.5) imply that the torque equilibrium cannot be obtained with three torques applied in the clockwise direction as shown in Figure 7.12. If Te is applied counter-clockwise, equilibrium is obtained.
Summing Equations (7.4) and (7.5) leads to:
(7.6)
which is an obvious conclusion since the PSD system is at equilibrium under the action of the external torques. In terms of engine and motor torques:
7.3.1.1 Speed Constraints
Equation (7.2) is the governing relation between the three speed components in a simple PSD system. These three speeds are in fact physical parameters of the vehicle motion and are related to the engine, motor and vehicle speeds. It is clear that Equation (7.2) is acting like a constraint on the speeds of the main vehicle components and does not allow each speed component to vary independently.
On the other hand, the engine and two motors/generators each have speed limitations. The engine can work only in one direction from idle speed up to its limiting speed, whereas the two motors/generators work in both directions from their lower (negative) up to upper (positive) limiting speeds.
The constraint imposed by Equation (7.2) is representative of an inclined line connecting the three speeds ,
and
placed at relevant geometric locations. Figure 7.13 illustrates this geometry together with limitations for the speeds of components. The three vertical lines from left to right represent the axes for the speeds of MG1, engine and MG2 respectively, drawn at relative distances proportional to NR and NS. Two possible working lines are included in Figure 7.13 to clarify the concept. The following example uses numerical values for the parameters.
Figure 7.13 Speed constraints in a simple PSD system

As the speed of the MG2 can vary continuously, the vehicle speed which is proportional to this speed will also vary accordingly. Therefore, this type of PSD acts like a continuously variable transmission (CVT).
Example 7.3.1
In a PSD the tooth numbers for the ring and sun gears are 78 and 30 respectively. The ring gear is directly connected to the shaft of MG2 and to the driving wheels through a reduction gear with overall ratio of nf = 4. For a wheel radius of 30 cm, find the speeds of MG1 and MG2:
a. if the vehicle is at rest and the engine is off;
b. if the vehicle is at rest and the engine is at idle (1000 rpm);
c. if the vehicle is moving forward at 60 km/h and the engine is at idle;
d. if the vehicle is moving forward at 160 km/h and the engine is at 4000 rpm.
Solution
According to Equation (7.2), the relation of MG1 rotational speed with engine and MG2 speeds is:
On the other hand, the speed of MG2 is directly related to the vehicle speed:
Substituting in the first relation and using the given information results in:
in which and v are in rpm and km/h respectively. The numerical results for the four cases are summarized in Table 7.3.
Table 7.3 Numerical results of Example 7.3.1
This constraint causes some limitations on the operation of components, for example, the engine, as it forces them to work at a specific speed. For instance, at low vehicle speeds, the engine cannot work at its highest speed. The speed limit for the engine is dictated by the speed of the MG1. At the highest speed for the MG1, the engine speed will be located below its maximum speed as shown in Figure 7.14. The relation for this limiting speed is:
(7.8)
Figure 7.14 Speed limit for engine at low vehicle speeds

7.3.1.2 Power Flow
It is a simple task using Equations 7.2 to 7.5 to show that:
or simply:
This demonstrates the conservation of power and at the same time the power splitting feature of the device. The power received by the vehicle is the summation of the engine and battery power minus the lost power:
Assuming no power losses (the inclusion of power losses is considered in Problem 7.2), the total power delivered to the wheels is the summation of PSD output and MG2 power (see Figure 7.4):
(7.12)
The minus sign for PR is necessary to adjust its torque sense with its rotation. Substituting from Equation (7.10) will result in:
(7.13)
According to Equation (7.11) if the vehicle power PV is larger than the engine power Pe, the battery is also transmitting power through MG1 or MG2 (Equation (7.14)). Conversely, if Pe is larger than PV, then MG1 or MG2 act in generator mode and receive power from the engine to recharge the battery. It is important to note that we have assumed the PSD output shaft (ring gear R) is directly connected to MG2 and thus only the speed of MG2 is identical to the output speed of PSD and its torque T2 is independent of PSD output torque TR.
Example 7.3.2
For the vehicle in Example 7.3.1, the resistive force on a level road is given by (v in m/s). The engine is working at full throttle and its torque-speed curve is given by:
For the case (d) in Example 7.3.1, assume the vehicle is at a steady motion.
a. Calculate the torque and power of each component.
b. Specify the direction of flow of power in the system.
Solution
a. Since the speed is steady, the torque acting on the common shaft of the ring gear and MG2 at the given speed is:
From the engine torque equation at the given rpm we obtain Te = 110.84 Nm. From Equation (7.5) the PSD output torque can be determined:
The minus sign indicates that the direction of engine rotation is opposite that of MG2. The torque of engine is larger than what is needed and the extra torque at output shaft (80.051 − 59.537 = 20.514) is absorbed by MG2. The torque of MG1 from Equation (7.3) is −30.79 Nm.
The power of each component is simply its torque multiplied by its speed:
Since the rotating direction of MG2 is different from its torque direction, it is in generator mode and has a negative power.
b. Vehicle power demand PV is equal to: .
The engine power is greater than the demand power, therefore, the battery is charged with power PB = PV − Pe = −11,147 W (Equation (7.11)). In fact, MG2 takes 12,156 W of engine power and delivers it to the battery. MG1, on the other hand, works in motor mode and receives 1,009 W of battery power. The balance of power towards the battery, therefore, is PB = −12,156+1,009 = −11,147 W as Equation (7.14) indicates.
7.3.1.3 Power Circulation
In the ‘engine-only’ mode no power is received from the battery, nevertheless owing to speed dependencies through the PSD, the engine power cannot be delivered directly to the driving wheels. In such circumstances MG1 and MG2 will either work as generators or motors. This causes mechanical power to be transformed into electrical power and back again by the two electrical machines. In Example 7.3.2 we observed the circulation of 1009 W of power.
Figure 7.15 illustrates two possible cases for the power circulation in ‘engine-only’ mode. In Figure 7.15a a fraction of the mechanical power from the engine is taken by MG1 and converted to electrical energy that is received by MG2 and converted in mechanical energy and delivered to the driving wheels. Figure 7.15b shows the power circulation in the opposite direction.
Figure 7.15 Power circulation

For the cases of ‘engine-only’ mode shown in Figure 7.15a, the circulating power PCir is equal to the power of MG1 for case (a) and the power of MG2 for case (b). If no power loss is considered for this mode of operation, then PB vanishes and from Equation (7.14):
(7.15)
Making use of Equations (7.5) and (7.9) will result in:
The variation of power circulation ratio PCir/Pe versus speed ratio ω2/ωe for the gear numbers of Example 7.3.1 is depicted in Figure 7.16.
Figure 7.16 Variation of power circulation ratio with speed ratio

It is obvious that in practice, power circulation will generate power loss due to inefficiencies involved in converting between mechanical power and electrical power.
Example 7.3.3
For the vehicle in Example 7.3.2 with 1500 kg mass, the engine is working at full throttle and the vehicle is accelerating at a speed of 120 km/h. Assume no power losses and for ‘pure-engine’ mode:
a. Calculate the vehicle acceleration.
b. Determine the power circulation of the system using component powers.
c. Determine the power circulation using Equation (7.16).
Solution
a. For maximum acceleration, the engine must work at maximum torque. From the engine torque equation we obtain: Te = 111.7 Nm and ωe = 3562 rpm.
Since no power is taken from the battery, the total power demand of the vehicle is produced by the engine, thus: . Vehicle acceleration therefore is:
b. The torque acting on the common shaft of ring gear and MG2 is:
According to the procedure explained in Example 7.3.1, the torques and speeds are obtained as:
The output torque of the PSD (TR) is less than the total output torque (TO). The balance is the torque of MG2:
The component powers are:
which indicates that MG1 and MG2 are acting as the generator and motor respectively.
c. The speed ratio in Equation (7.16) is 1.1916 and the right-hand side is:
7.3.1.4 Maximum Driving Force
Assuming sufficient friction is available at the tyre contact area, the driving force of the vehicle results from the torque available at the driving wheels. The maximum torque delivered to the wheels is the summation of torques generated at the PSD output (TR) and MG2 torque (T2) multiplied by their gear ratios. Therefore, the torque delivered to the driving wheel with different gear ratios n1 and n2 for PSD output and for MG2 (see Figure 7.5) is:
(7.17)
The output of the PSD in turn is the result of interaction between the engine torque and MG1 torque. Using Equation (7.5), we obtain:
(7.18)
For the case when the PSD output and MG2 are both on a single shaft, the available driving force is:
(7.19)
Depending on the mode of vehicle operation, therefore, the maximum traction force varies. In battery-only mode, the engine is off and only T2 will be present in Equation. In engine-only mode, however, due to power circulation, the T2 term will still be present.
Example 7.3.4
The vehicle of Example 7.3.3 is accelerating at full throttle from a standstill with an engine idle speed of 1000 rpm. Other necessary information is provided in Table 7.4.
Table 7.4 Additional data for Example 7.3.4
Assume no power loss and for ‘engine-only’ mode, plot the variation of vehicle speed, acceleration, traction force, component torques and component powers versus time.
Solution
Solving this problem requires a large number of calculations and therefore it is helpful to write a program for it. The following comments are useful before starting to solve the problem:
1. Although the vehicle is in engine-only mode, the circulating power is present and both motor/generators are involved.
2. The initial condition of zero vehicle speed results in a zero speed for MG2 and thus at the start of calculations, MG2 cannot absorb and deliver the circulation power to the wheels. It can be assumed that the traction force is at its maximum at time zero.
3. Assumption of no power loss lets us use at every instant (note that vehicle is in ‘engine-only’ mode).
4. ω2 (=ωm) is directly related to vehicle speed, whereas ω1 must be determined from the circulating power (note that the circulating power is also equal to MG1 power).
A MATLAB program is provided for the solution of Example 7.3.4 with listings given in Figures 7.17 and 7.18. The solution involves numerical integration to obtain the vehicle speed and for this reason a function named ‘v_find’ is shown in the main program. The comments are included in the programs to make them self-explanatory. Results are plotted in Figures 7.19–7.23
Figure 7.17 Main MATLAB® program listing for Example 7.3.4
Figure 7.18 MATLAB function program listing
Figure 7.19 Variations of vehicle speed and acceleration
Figure 7.20 Variation of total vehicle tractive force
Figure 7.21 Variations of component torques
Figure 7.22 Variations of component speeds
Figure 7.23 Variations of component powers
7.3.2 EM Compound PSD
The PSD system of Section 7.3.1, despite the simple construction, imposes limitations on the power management and operation of the vehicle. In Section 7.2.3.2 several types of PSDs were discussed. The objectives of new designs are to introduce more flexibility to the operation of the hybrid system and power flow schemes.
A PSD design, developed at Iran University of Science & Technology (IUST), is a compact device that is based on a planetary gear set and incorporates an additional input shaft for MG2. The layout of this device is called easier management or EM PSD (EM also stands for Emadi-Mashadi) is depicted in Figure 7.24 (also see [2]). An input shaft ‘N’ connected to a second ring gear is the additional feature of the simple planetary gear set.
Figure 7.24 Layout of EM compound PSD

Kinematic relationships for this device can be written as follows:
in which:
(7.23)
(7.24)
Graphical representations of Equations (7.20) and (7.21) can be seen in Figure 7.25 for a PSD layout with kP < 1 (like Figure 7.24). The two left and right vertical lines and the engine line constitute the Equation (7.20) which is exactly equal to Equation (7.2) of the simple PSD system. The three right-hand side vertical lines, on the other hand, represent the relation defined by Equation (7.21).
Figure 7.25 Speed constraints in EM PSD system

For values of kP larger than unity, the two right-hand side vertical lines change over. At kP = 1, the EM-PSD will be identical to the simple PSD.
Example 7.3.5
Use the information of Example 7.3.1 and find the speeds of MG1 and MG2 for the four cases of the same example. Obtain the results for two cases with the radius ratio of small planet gear to the larger planet gear of kP = 0.75 and kP = 1.5.
Solution
Similar to Example 7.3.1, the speed of vehicle is directly related to the speed of PSD output which in this case is the ring gear R. The rotational speed of the ring gear is:
According to Equations (7.20) and (7.21), the relations of MG1 and MG2 speeds with engine and ring gear speeds are available. So once the speed of ring gear from vehicle speed is calculated, the required speeds are easily found. The numerical results for the four cases are summarized in Table 7.5.
Table 7.5 Numerical results of Example 7.3.5
It can be seen that the speed values of MG1 are identical with those of Example 7.3.1.
7.3.2.1 Torque and Power
The quasi-static torque equilibrium of the device is of the form:
Assuming no power loss in the device results in:
(7.26)
Substituting from Equations (7.20) and (7.21) into Equation (7.25) leads to:
(7.27)
Then by using Equation 7.25, one obtains the following equations for the torques of MGs:
The total power demand from the vehicle motion is produced by the engine and the battery and in this case is only delivered through the main ring gear (see Figure 7.24):
Substituting from Equation (7.25), the result is:
(7.31)
which leads to:
(7.32)
This confirms the fact that the exchange of power with the battery is performed by MG1 and MG2 together.
Example 7.3.6
For the vehicle and PSD of Example 7.3.5, repeat Example 7.3.2 and at a steady motion for the case (d) of Table 7.5:
a. Calculate the torque and power of each component.
b. Specify the direction of flow of power in the system.
Solution
a. The torques acting on the shaft of ring gear and the engine torque at the given speed for both cases of kP are TR = 59.537 Nm and Te = 110.84 Nm (see Example 7.3.2).
From Equations (7.28) and (7.29), the MG1 and MG2 torques can be obtained. The power of each component is found by simply multiplying its torque by its speed. The engine power is Pe = 46.429 kW.
The other results are provided in Table 7.6.
b. Vehicle power demand PV is equal to for both cases.
Table 7.6 Numerical results of Example 7.3.6
The engine power is greater than the demand power, therefore, the battery is charged with power PB = PV − Pe = −11,147 W (Equation (7.30)). In fact, MG2 takes part of the engine power and delivers it to the battery. MG1, on the other hand, works in motor mode and receives power from the battery. The balance of power to the battery, therefore, is: PB = PMG1+PMG2 = −11,147 W (a negative sign means the battery receives power).
7.3.2.2 Power Circulation
In ‘engine-only’ mode the power source is only from the engine while the power is delivered to the output through the ring gear R only. Thus, if power loss is ignored:
(7.33)
Equation (7.34) represents the power circulation between MG1 and MG2 during ‘engine-only’ mode of vehicle motion, that is:
(7.35)
Making use of Equations 7.20, 7.28 and 7.33 (or Equations 7.21, 7.29 and 7.33), one finds the following expression for the circulating power as a fraction of engine power:
In general, at two points with speed ratios of either or
, the circulating power vanishes. The former point depends only on parameter m that is dependent on the layout of original epicyclic set (see Equation (7.22)). This point with a speed ratio of greater than unity is always physically possible. The latter point is dependent on the relative sizes of planet gears in the EM-PSD and for values of n < 1 will lie on the negative side of speed ratio axis and will not be present in forward vehicle motions. n = 1 refers to the case when the two PSD systems are identical.
The variation of power ratio of Equation (7.36) with the variation of speed ratio for different values of parameter n is plotted in Figure 7.26. The parameter m is taken equal to 2.6 that refers to tooth numbers NS = 30 and NR = 77. It is clear that for values of n >1 the circulating power is reduced considerably in some working regions compared to the original PSD system.
Example 7.3.7
For the vehicle and conditions of Example 7.3.3 and the PSD of Example 7.3.5, determine the power circulation of the system.
Solution
The vehicle acceleration is 0.45 m/s2 with Te = 111.7 Nm and ωe = 3562 rpm. The torque acting on the output shaft (main ring gear) is 93.737 Nm (see Example 7.3.3).
According to procedure explained in Example 7.3.3, the results are obtained and summarized in Table 7.7 for both kP values.
Table 7.7 Numerical results of Example 7.3.7
Figure 7.26 The variation of circulating power ratio versus speed ratio for EM-PSD

7.4 HEV Component Characteristics
Hybrid electric vehicles employ electric motors either to power the vehicle in pure-electric mode or to assist the IC engine during brief periods of high torque demands. On the other hand, electric generators are used to recapture the energy of a vehicle during braking to recharge the battery. A substantial advantage of a hybrid vehicle, however, is the optimum use of its power-generating components. IC engines and electric machines each have their own operating characteristics that influence the whole performance and efficiency of an HEV. In hybrid vehicle designs, therefore, maximizing the overall efficiency and reducing the vehicle emissions are major goals.
7.4.1 The IC Engine
The internal combustion engine is still a dominant source of power in a hybrid electric vehicle. The influential characteristics of an engine include its torque-speed performance, efficiency behaviour and pollution. The performance characteristics of IC engines were discussed in detail in Chapter 2 and fuel consumption issues were analyzed in Chapter 5. Those readers who are familiar with materials covered in Chapters 2 and 5 will have no difficulty in following the subject of this chapter.
7.4.2 Electric Machines
Electric machines are well-established components in conventional vehicles as starters and alternators, for cranking of the engine and producing electricity to charge the battery. In HEVs, however, the electric machines play more important roles as they deliver greater power for extended durations.
Different electric machines depending on their construction will have different characteristics. Two key features of electric machines from a HEV design point of view are their torque-speed and efficiency characteristics. Various types of DC or AC machines have been developed and each type has found its best place in the industry. The range of electric motors used in HEV designs includes separately excited DC motors, permanent-magnet synchronous and induction AC motors, brushless DC (BLDC) and switched reluctance motors.
In general, AC motors are less expensive and maintenance-free, but they require more sophisticated power electronics. But owing to higher power density and higher efficiency of AC motors, these types of motors are used in the majority of HEV applications.
7.4.2.1 Motor Torque
The distinctive property of electric motors compared to IC engines is that they produce torque from zero speed. The torque-speed characteristics of different types of motors in general depend on their construction, but they typically contain two phases of constant-torque and constant-power controlled by using power electronics.
Figure 7.27 shows a typical variation of motor torque and power with shaft speed. With increasing supply voltage to the motor while the flux is kept constant, the motor speed increases and a high constant torque is obtained. At the point where the motor voltage equals the source voltage, the constant-torque phase ends and during the second phase, at that constant voltage the flux decreases and constant maximum power is maintained. This feature is very useful when the motor is used as a vehicle traction motor since it can produce large driving forces at low vehicle speeds (even at zero speed and without needing a clutch) and can produce high acceleration up to the maximum available power of the motor.
Figure 7.27 Typical maximum performance curves of HEV electric motors

7.4.2.2 Motor Efficiency
An electric motor receives electric power and converts it to mechanical power. In any physical system the output power is always less than the input power due to power losses through the energy flow components. According to Figure 7.28a part of the electric input power PE will be lost all the way through the inverter to the motor and inside the motor itself. Defining this power loss PL, the output mechanical power PM will be:
Figure 7.28 Power flow from battery to motor output

(7.37)
The motor efficiency ηM at every working condition is defined as the ratio of output power to input power:
(7.38)
The power loss, therefore, can be expressed in terms of input power:
When the motor efficiency is determined through laboratory tests, it turn out that the efficiency is dependent on the working condition of the motor and is not a constant value. In a mathematical representation:
(7.40)
In the motor T-ω plane, the efficiency of each working point has a discrete value. There will be several points with an identical efficiency value, so that when these points are connected to one another, an iso-efficiency curve will appear. Repeating this process for other equal efficiency points, generates an ‘efficiency map’ for the motor. A typical efficiency map for an electrical motor is provided in Figure 7.29.
Figure 7.29 A typical motor efficiency map

For a generator, the power direction of Figure 7.29 reverses and the electric power received at battery terminals is:
(7.41)
where is the power loss in generator mode. Similar to the motor, the efficiency is defined:
(7.42)
If it is assumed that the power loss is independent of the direction of power flow, then equating the right-hand sides of Equations (7.39) and (7.43) leads to:
Example 7.4.1
Table 7.8 includes efficiency figures obtained for an electric motor in its motoring phase of operation. The intention is to use this information and generate similar result for the generating phase of the same motor. Use Equation (7.44) and build efficiency plots for generating phase.
Table 7.8 Motor efficiency values of Example 7.4.1
Solution
A MATLAB program is provided for the solution of this example. The program listing is given in Figure 7.30. The main part of solution makes use of MATLAB ‘contour’ function. The comments are included in the program to make it self-explanatory and the resulting plot can be seen in Figure 7.31.
Figure 7.31 Efficiency maps of Example 7.4.1

7.4.3 The Battery
The battery is a critical component central to the success of electric and hybrid vehicles. Large power demands from the vehicle battery and high life expectations make it a very complicated system that requires instantaneous monitoring and management. The battery pack of an HEV is constructed by wiring battery cells typically in series to generate a high voltage for electric motor drive, but to develop higher currents the cells are sometimes also wired in parallel. The battery pack also comprises a number of sub-components including sensors for voltages, current, and temperature; a thermal conditioning system; a battery management system and power electronics to isolate the battery pack.
The two most important characteristics of a battery are its energy capacity and efficiency. The energy capacity of a battery is essential when long distance electric driving is concerned. Of course it is always possible to use larger battery packs but obviously this will at the same time increase the weight and cost. Therefore, the ‘energy density’ of a battery, defined as the ratio of its energy capacity to its weight, is a very important factor.
The battery capacity Q can be defined as the amount of free charge generated inside the battery and is measured in amp-hour (Ah). It represents the capability of the battery to generate electric current within a time span (e.g. an hour). Such electric current is called the discharge rate of the battery. The capacity may also be given in Watt-hour (Wh) which is the battery's Ah multiplied by its voltage.
7.4.3.1 State of Charge
The battery cell is a delicate system that can be permanently damaged if mistreated. Over-charging or over-discharging a battery cell may cause damage and result in reduced life. In order to avoid damage to the battery, therefore, it is necessary to estimate its present charge condition to assess how much it could be charged or discharged.
The state of charge (SOC) of a battery is a measure of the present charge capacity of the battery. It is the amount of capacity that is left after starting to discharge from a fully charged condition. What is meant by SOC is an indication of the fraction of charge remaining in each cell, and is measured in percentage (%) of the cell's full capacity. With an accurate measure of SOC, cell damage can be avoided by controlling the current. There is no sensor available to measure SOC directly and thus it must be estimated. In mathematical form, the instantaneous SOC can be expressed as:
in which Q0 is the fully charged capacity and I(t) is the instantaneous discharge current of the battery. is positive during discharging and negative during charging.
7.4.3.2 Battery Efficiency
A battery, like every physical system, loses some energy during power flow. The battery power loss is modelled by an ohmic power consumed by an internal resistance Ri shown in Figure 7.32. For a battery current I the loss is:
Figure 7.32 The internal resistance of a battery

Figure 7.33 Typical variation of battery internal resistance

(7.46)
The open-circuit voltage of the battery VO is related to the close-circuit discharging voltage Vd by writing KVL for the discharging circuit of Figure 7.32:
(7.47)
Multiplying both sides by the current I and noting that the VdI term is the output power, results in:
(7.48)
The efficiency of discharging can be defined as the ratio of output power to input power, or:
in which Rd is load resistance during discharging:
(7.50)
Rd can be obtained by measuring the terminal voltage and current values.
In the charging phase, the direction of the current in Figure 7.32 reverses and the charging voltage Vc is higher than the open-circuit voltage. Following a similar procedure, for the efficiency of battery during charging, one can find:
(7.51)
with Rc defined similarly as:
(7.52)
The internal resistance Ri of a battery is not a constant value and is different during charge and discharge phases. It also depends on the amount of capacity of charge (SOC) of the battery. A typical variation of a battery's internal resistance with SOC for charging and discharging phases is shown in Figure 7.33.
Example 7.4.2
The variation of battery internal resistance with SOC during charging and discharging phases is given (in Ohm) as:
Plot the efficiency maps of the battery in a power-SOC diagram by assuming a constant terminal voltage of 280 V.
Solution
At a given power P the current and load resistance simply are:
For both cases of discharging and charging the two parameters have identical values at specified terminal voltage and power.
A MATLAB program with listing given in Figure 7.34 is provided for the solution of this example. The values of the above parameters are evaluated at 150 points for each charging/discharging phase for power values of 0 to 15 kW. At 10 SOC values of from 0 to 100% the efficiency of battery is evaluated using Equation (7.49) (or 7.51). Making use of MATLAB ‘contour’, the contours of battery efficiency are drawn and the resulting plot is shown in Figure 7.35.
Figure 7.35 Battery efficiency map of Example 7.4.2
Example 7.4.3
A series hybrid vehicle of 1000 kg mass uses a battery pack of 150 V. The vehicle with a fully charged battery starts a driving cycle as shown in Figure 7.36.
Figure 7.36 Driving cycle of Example 7.4.3
A motor with the efficiency curve given in Figure 7.31 is driving the car via a single reduction gear. The vehicle speed is related to the motor speed by a factor of 0.03. The resistive forces acting on the vehicle can be obtained from (v is the speed of the vehicle in m/s).
If the battery initially has a charge value of Q0 = 50 Amp-h, max recharge current is 20 A and the efficiency of charging/discharging is 0.9 (constant):
a. Derive equations for the motor speed, motor torque, motor power, battery current and battery SOC versus time.
b. When the vehicle follows the driving cycle, plot the variations of parameters in (a) for different parts of the cycle.
Solution
a. At a given vehicle speed v the motor speed is given by
The motor torque is related to the traction force of the wheels by . The traction force equals the resistive force plus acceleration force:
.
The motor output power is simply its torque multiplied by its speed.
The input power to the motor (electric power) is its output power plus the lost power:
The latter is found from the motor efficiency map, knowing the motor speed and torque. The battery current is obtained from electrical power demand of the motor divided by the battery voltage. The battery SOC can be obtained using Equation (7.45) and by integrating the battery current.
b. A MATLAB program with listing given in Figure 7.37 is suitable for this example. The values of the above parameters are evaluated at several points for each 0.1 second of driving cycle. The program of Example 7.4.1 is also utilized for the generation of motor efficiency values.
Figure 7.37 MATLAB listing of Example 7.4.3
Figure 7.38 Motor output torque of Example 7.4.3 during one cycle
Figure 7.39 Motor output power of Example 7.4.3 during one cycle
Figure 7.40 Battery current of Example 7.4.3 during one cycle
Figure 7.41 Battery SOC of Example 7.4.3 during one cycle
For the deceleration parts of the driving cycle, it is assumed that the motor goes into generator mode and recharges the battery with a maximum of 20 Amps.
The resulting plots for the variations of different parameters in a single driving cycle are shown in Figures 7.38–7.41.
7.4.3.3 Battery Management System
The battery pack is an expensive component of the hybrid vehicles and it is important to prolong its life by implementing proper management schemes. There are several factors affecting battery performance and life. The discharge strategy of a battery is of particular importance as the performance of the batteries is reduced if they are deeply discharged regularly. The battery performance is highly dependent on temperature and different battery types work best within specified ranges of temperatures.
A battery pack consists of several battery cells stacked in series. The number of the battery cells in a pack increases the total voltage of the overall pack. To develop a voltage of 400 V using 4.2 V cells, a stack of 96 cells is necessary. When batteries are used in series, the state of health of every single cell will be important because the malfunctioning of any of the cells will lead to the failure of the entire battery pack. Moreover, if one cell in a pack has slightly less capacity than the other cells, then its state of charge will gradually deviate from the rest of the cells over multiple charge/discharge cycles. This will eventually cause deep discharge of that particular cell and thus permanent damage. To prevent this from happening, each cell's voltage must be monitored to determine its state of charge. If the SOC of a cell is found to be out of balance, it should be individually charged (or discharged) to balance its state of charge with other cells.
An automotive battery management system (BMS) has to work in real time in a rapidly varying environment involving continuous charging and discharging. Battery lifetimes for automotive applications exceed 10 years and the BMS must carefully control and monitor the batteries. Hybrid vehicle batteries require both high power charge capabilities for regenerative braking and high power discharge capabilities for the launch assist or boost. For this reason, their batteries must be maintained at a SOC that can discharge the required power but still have enough headroom to accept the necessary regenerative power without risking overcharging the cells.
The BMS has two major tasks: (1) protecting the cells; and (2) keeping the individual cells in optimum SOC. One of the prime functions of the BMS is to provide the necessary monitoring and control to protect the cells from going into out of tolerance operating conditions. Battery overheating is one example of these for which the cooling fans can be turned on and if the overheating becomes excessive, then the battery must be disconnected. Since over-charging and under-discharging are two of the prime causes of battery failure, determining the SOC of each individual cell in the battery pack is necessary to check for uniform charge in all of the cells in order to verify that individual cells do not become overstressed.
If the HEV battery is fully charged, the capability for charging during regenerative braking will be reduced and hence braking efficiency will be diminished. On the other hand, deep discharging damages the battery and must be prevented. Therefore, two upper and lower limits for the battery SOC are defined. The lower limit is set to prevent over-discharge which shortens the life of the battery and the upper limit is set to leave some room for absorbing the kinetic energy of the vehicle during braking. Typical values for the battery operating ranges shown in Figure 7.42 are different for EVs, HEVs and PHEVs due to their different usage in the vehicle. For EVs and PHEVs, the working range is typically as low as 20% and as high as 95%. For HEVs, the minimum working SOC ranges from 40% up to 60% and the maximum working SOC ranges from 60% up to 80%. For SOC values below the working range, no discharging is allowed and the vehicle is unable to work in electric mode. If the SOC value is higher than the high working value, the battery is never charged by the engine but it may be charged through the regenerative braking.
Figure 7.42 Battery operating ranges

Typical driving conditions of a vehicle are very diverse and cannot therefore be fully anticipated. In particular, normal driving of a vehicle includes extensive use of part load conditions that are not easy to model. Special full load maximum performance cases, however, are simpler to model.
The performance and operation of hybrid electric vehicles, on the other hand, greatly depend on the type of hybridization. The performance of a series hybrid vehicle is basically dependent on the characteristics of its electric motor. The operation of a parallel HEV at the three major modes of pure engine, pure electric and hybrid, however, is influenced by the way power is controlled and managed.
7.5.1 Series HEV
The driving performance of a series hybrid vehicle is governed by its electric motor characteristics. The traction force produced at the driving wheels is the result of the motor torque Tm amplified by the reduction gear ratio n:
(7.53)
in which is the efficiency of driveline from motor to the wheels and rW is the effective radius of the driving wheels. The typical full load performance of a motor was shown in Figure 7.27 in which two regimes of constant torque and constant power were present. Propelling the vehicle in its full performance is achieved when the motor follows its full load curve. The whole performance of the vehicle can be divided into two separate regions of constant torque and constant power. The governing equations will be different at each phase.
7.5.1.1 Constant Torque Phase
With reference to Section 3.6, the speed of the vehicle starting from rest during the constant torque phase can be described by:
(7.54)
where:
(7.55)
The maximum speed at the end of constant torque phase is where the motor is at its base speed ωb:
The time to reach this speed can be obtained from following relation:
The distance travelled during this constant torque period is:
(7.58)
It is obvious that at time tb the maximum power of motor is utilized and after this point the power remains a constant value Pmax:
(7.59)
that is, proportional to the base speed ωb of motor or the base velocity vb of the vehicle. In other words, for higher base velocities, the motor power must be larger. On the other hand, a higher base velocity means a longer base time tb which means the high acceleration of the vehicle lasts longer. In terms of vehicle performance, this is a good factor but it can only be achieved at the expense of larger motor powers.
7.5.1.2 Constant Power Phase
The governing equations for the constant power phase of the vehicle motion can be obtained by making use of the results of Sections 3.5.4 and 3.5.8. The maximum vehicle speed can be determined by using:
in which:
(7.61)
The time to reach a desired speed v can be obtained from the equation below:
in which C is the constant of integration and must be determined from an initial condition. The coefficients k, k1 and k2 are found from:
(7.64)
(7.65)
Theoretically, the time to reach the maximum speed is infinity. A reasonable time can, however, be obtained for the time to reach very close to the final velocity (e.g. 98% of it) by first obtaining the constant C for the initial condition of vb at tb (i.e. end of constant torque phase), and then substituting a desired value for v (e.g. 0.98 of vmax) into Equation (7.63).
Example 7.5.1
An electric motor used in an EV has a maximum torque of 124 Nm up to the base speed of 2200 rpm. The vehicle information is given in Table 7.9:
Table 7.9 Vehicle information for Example 7.5.1
a. Plot the motor torque-speed diagram.
b. Determine the base velocity and maximum velocity of the vehicle. Also specify the motor speed at the maximum speed.
c. Verify the answer of (b) by plotting the tractive and resistive forces in a single diagram.
d. Determine the base velocity time and the times to reach 98%, 99% and 99.5% of the maximum vehicle speed.
Solution
Direct use of the available equations can lead to the required information. Due to the complex formulae, however, a MATLAB program will be useful. This program is listed in Figure 7.43.
a. The motor torque-speed plot consists of two constant torque and constant power parts. The first part can be constructed simply by the information given for the maximum torque and base speed. The constant power in the second part is obtained by multiplying the maximum torque by the base speed. The torque at any given speed then is the value of the constant power divided by the speed (in rad/s). The result is shown in Figure 7.44.
b. The value of base speed obtained from Equation (7.56) is 13.8 m/s. The final velocity is determined by using Equation (7.60) and the result is 36 m/s. The motor speed at the maximum vehicle speed will be 600 rad/s (5730 rpm).
c. The intersecting point of the force diagram of Figure 7.45 verifies the result of part (b).
d. From Equation (7.57) the time of base speed is 9.8 s. Equations (7.63)–(7.66) are used to estimate the time to reach the maximum speed by evaluating the speed at 0.98, 0.99 and 0.995 of the maximum speed. The results are 88, 104.7 and 121.4 s.
Figure 7.44 The torque-speed diagram of the motor
Figure 7.45 Maximum speed at intersection point of tractive and resistive forces
7.5.2 Parallel HEV
The performance of a parallel HEV depends primarily on its mode of operation. In the electric-only mode, the performance is similar to that of a series HEV discussed in Section 7.5.1. In the engine-only mode, the performance is similar to that of a conventional vehicle discussed in detail in Chapters 3 and 4.
In the hybrid mode, the performance can be analyzed depending on the control strategy that specifies the power distribution of the two sources of energy. The maximum performance of a parallel hybrid vehicle is obtained when both the ICE and motor simultaneously produce their maximum torques to propel the vehicle. The resulting traction force depends on the gear ratios of both the engine and the motor:
(7.67)
where n is the gear ratio from the prime mover to the driving wheel (subscripts e and m stand for engine and motor respectively). In the following example the performance of a parallel HEV will be examined.
Example 7.5.2
A parallel HEV with the information given in Table 7.10 uses a motor with specifications given in Example 7.5.1. The engine WOT curve is of the form:
Table 7.10 Vehicle information for Example 7.5.2
The motor output is directly connected to the final drive whereas the output of ICE is connected through a five-speed transmission with gear ratios 4.0, 2.8, 1.85, 1.25 and 0.85.
For a maximum driving performance of the vehicle:
a. Plot the ICE and motor torque-speed diagrams in a single figure.
b. Plot the total tractive force diagram of the vehicle against the forward speed. Also include the resistive force curve and find the maximum vehicle speed.
c. Find the final speed of the vehicle for electric-only mode by using the method described in Example 7.5.1.
d. Obtain the final speed of the vehicle at engine-only mode by plotting the tractive-resistive force diagrams. Also include a diagram for electric-only mode.
Solution
A MATLAB program with the listing of Figure 7.46 is prepared for this example.
Figure 7.46 The MATLAB listing of Example 7.5.2
a. The motor torque-speed variations are similar to that of the previous example with the exception that its speed is taken up to 6000 rpm. The engine torque-speed variation is calculated from the given formula. The result is shown in Figure 7.47.
b. The tractive force resulting from the engine torque will be different at each gear. The speed of the vehicle at each gear varies according to the revolution speed of the prime movers. The total tractive force at each gear is obtained simply by summing the engine and motor parts. The result is plotted in Figure 7.48. The intersecting point of the tractive force diagram with the resistive force curve in gear 5 indicates the final speed of 198.5 km/h. Note that at gear 1 it is assumed that the traction force at zero speed corresponds to the engine torque at a speed of 1000 rpm.
c. This part is exactly similar to the previous example and thus has been excluded from the program listing. The result is 34.8 m/s (125.4 km/h) for the maximum speed in electric-only mode.
d. A force diagram for the engine-only mode is shown in Figure 7.49 at gear 5. The maximum vehicle speed is 156 km/h. In the same plot the motor traction diagram is also included. The result of case (c) is verified.
Figure 7.47 The ICE and motor torque-speed diagrams
Figure 7.48 Total tractive force at different transmission gears
Figure 7.49 Maximum vehicle speeds in engine-only and electric-only modes
One of the critical choices in the hybrid vehicle designs is the relative size of the IC engine and the electric motor. Using a large electric motor will require more electric power and thus requiring a larger, heavier, and more expensive battery. The size of components, on the other hand, depends on the performance requirements; for example, if a long electric range is needed, using a large battery is inevitable, or to obtain high acceleration during the electric-only mode, a large electric motor must be chosen.
Another decisive factor in the selection of components for an HEV is the overall cost and the fuel economy of the vehicle. Therefore, a good design is the one with the required performance at lower cost and fuel consumption.
7.6.1 General Considerations
The design of an HEV is a complicated task that requires a multidisciplinary knowledge of several relevant sciences. In this section, we intend to discuss the general design issues briefly. In order to get a general understanding of the differences between the conventional and hybrid powertrains, let us take an example of converting a conventional vehicle to a hybrid electric vehicle. The major changes include adding at least one electric motor and related mechanical devices together with a large battery pack to the base vehicle. Several problems in such conversion include:
- insufficient space for the motor, the battery and control boxes;
- conflict between the existing controllers (e.g. ECU) and the new controllers;
- extra sensors and on-board displays are necessary;
- existing alternator cannot charge the new battery;
- extra mass is added to the vehicle.
In addition, there are some existing components that are not compatible with the new system such as the existing battery, starter motor and alternator. Also when electric-only mode of operation is selected, other unexpected difficulties will be faced, such as problems in braking due to loss of engine vacuum and booster function, loss of power assist in steering and loss of the air conditioning compressor.
This example indicates that the structure and configuration of an HEV are basically different from a conventional vehicle and thus it must be designed independently based on its own specifications and requirements. The critical design issues are discussed in the following section.
7.6.1.1 Downsized Engine
It is obvious that the desired performance for a vehicle requires a specific amount of power to be installed in the vehicle. When the electric motor takes a share of the installed power, then the engine can be downsized relative to its original size in a conventional vehicle. This will allow a smaller engine on an HEV with a lower size and weight to be used.
On the other hand, an IC engine has low efficiencies at low loads and high revolutions (see Chapter 5) and its optimum operation is restricted to certain regions. In an HEV, the use of the electric motor allows the engine to operate close to its most efficient points for a range of vehicle speeds and loads and in turn to improve the overall fuel economy and emissions. Obviously the engine alone cannot produce a performance similar to that of a conventional vehicle, but with the assistance from the electric motor both the performance and fuel economy could be improved.
7.6.1.2 Electric Accessory Drive
When the vehicle is powered in electric mode, the accessories typically driven by the engine must now be driven by other means. Typical accessories include alternator, steering pump, AC compressor and water pump. Apart from the water pump and alternator that must work when the engine is on, the others must be available on demand even when the engine is off. Therefore, in an HEV it is necessary to drive the accessories by electrical power.
7.6.1.3 Sizing
The sizing of HEV components can be approached from different viewpoints. Overall vehicle performance is one of the important measures for the design of components and thus the motor size must be suitable for this purpose. On the other hand, a vehicle with acceptable performance must at the same time have low energy consumption and emissions. In addition, the cost of a vehicle and its components have to be as low as possible. These factors are usually contradictory and thus the sizing of components involves some compromise decisions.
7.6.2 Sizing for Performance
The performance of vehicle is a key factor in its selection by the owner. One of the basic steps in sizing the components is to determine the minimum sizes to achieve the required vehicle performance. Four of the major performance requirements for the vehicle can be considered as:
- achieving a desired maximum speed vmax on the level road;
- achieving a desired maximum speed
on a specified slope θ;
- accelerating up to a desired speed vd during a desired time td;
- negotiation of a desired slope at low speeds.
The first three requirements are related to the installed power, whereas the fourth requirement is related to the available torque. More details on the sizing of major HEV components are discussed in following sections.
7.6.2.1 Series HEV
For the series HEVs, the electric motor is the only traction source and must be capable of producing all the performance characteristics such as the maximum required acceleration and speed. Based on such requirements the overall characteristics of the motor can be specified.
Size of the Motor
The desired performance characteristics for the vehicle were defined as four requirements for the vehicle speed, acceleration time and grading. The first criteria can be considered as having a desired steady speed vmax over a flat or sloping road. The motor must be able to achieve this performance by generating the necessary torque and power. The tractive or resistive power requirement Prfs for the required steady speed simply is:
(7.68)
where FR is the total resistive force comprising the rolling resistance, gravitational and aerodynamic forces (see Section 3.4):
(7.69)
In which θ is the slope angle. Therefore, the motor output power is:
in which ηd is the driveline efficiency.
Alternatively, the desired performance of the vehicle can be regarded as achieving a desired speed vd when accelerating from a standstill during a specified time td. Again it is the motor's responsibility to produce sufficient power Pma to accelerate the vehicle. Consider the torque-speed diagram of a typical electric motor shown in Figure 7.50. This desired time is the summation of two terms:
Figure 7.50 Required performance from a motor

Figure 7.51 Process to determine motor power for a given vb

in which tCT is the time elapsed in the constant torque phase and tCP is the time vehicle accelerated in the constant power phase (Figure 7.50). The two portions can be determined by using Equations (7.57) and (7.63) written as follows:
where:
(7.74)
Coefficients k, k1 and k2 in Equation (7.73) are all dependent only on the Pma of the motor, whereas β in Equation (7.72) is dependent on the Tmax of the motor. At the end of the constant-torque phase (i.e. at t = tCT) we have:
Thus β can be expressed in terms of Pma and vb:
(7.76)
Therefore if only vb is specified, for any required time td the motor power Pma can be determined in an iterative process using Equation (7.71) as the constraint. This is illustrated in Figure 7.51 starting with an initial estimate for the motor power and refining it by an iteration process using Equation (7.71) as the constraint.
Example 7.6.1
A series HEV is designed to perform its 0–100 km/h acceleration in 20 seconds. For the vehicle with the properties given in Table 7.11, plot the variations of Pma, the wheel torque TW, and the times tCT and tCP as vb varies from 5 to 25 m/s.
Table 7.11 Vehicle information for Example 7.6.1
Solution
A MATLAB program as listed in Figures 7.52 and 7.53 is prepared based on the process of Figure 7.51 and Equations 7.72 to 7.76. The main part of the program is the iteration process that is carried out in a separate function called ‘delta_t’ and invoked from the main program. This function returns with the final value of Pma that satisfies the constraint Equation (7.73) while the required speed vd occurs at the desired time td. The condition of motor maximum speed at vehicle maximum speed is used to determine the ratio of the reduction gear.
Figure 7.52 The main program listing of Example 7.6.1
Figure 7.53 The function of Example 7.6.1
The results of the program are those plotted in Figures 7.54 and 7.55. It can be seen that for the specified vehicle the motor power rating increases with the increase in the base velocity. On the other hand, the minimum power rating is obtained for the minimum base velocity (even zero). The wheel torque, however, is decreasing with the base velocity but it has a minimum at a certain point. The motor torque can be determined once the gear ratio of the reduction gear is selected.
Figure 7.54 The variation of motor power and wheel torque with base velocity vb
Figure 7.55 The variation of tCT and tCP with base velocity vb
Figure 7.55, on the other hand, shows the constraint implying the desired velocity occurs at 20 seconds.
The foregoing example showed that the motor properties can be determined if the base velocity is specified. In a real problem, however, even vb is an unknown quantity depending on the motor characteristics and cannot be specified before the motor is selected.
An alternative approach is to only consider a desired velocity vd for the vehicle performance and generate useful plots for the design parameters. In this method, for selected motor powers, any other parameter such as vb or tCT is varied and the other design parameters are determined. For the vehicle of Example 7.6.1 and for the same desired velocity of 100 km/h, the output results of this approach are depicted in Figures 7.56–7.59 for ng = 5.0. Figure 7.56 shows that for lower times, greater motor powers are required. From Figure 7.56, for a 20-second desired time of Example 7.6.1, all power ratings (33 kW and higher) seem to be selectable. The other important factor in selecting the motor, however, is its torque capacity and Figure 7.57 can be used for this purpose. For instance, if the motor torque is limited to 200 Nm, a motor with the minimum power of 33 kW cannot achieve less than around 22 seconds. The 43 kW motor for this torque limit of 200 Nm has a td of about 17 seconds. An interpolation between the two results gives a power rating of around 37 kW that can produce a desired time of 20 s. The base velocity for this motor is around 11 m/s and this result can also be verified using the results on Example 7.6.1 (Figure 7.54).
Figure 7.56 The plot of desired time vs vb at different power ratings

Figure 7.57 The plot of motor torque Tm vs desired time at different power ratings

Figure 7.58 The plot of motor torque vs its base speed at different power ratings

Figure 7.59 The plot of base velocity vs tCT at different power ratings

If the motor torque has lower limiting values, it is clear that low required time values cannot be achieved. To resolve this problem, larger reduction gears must be used.
Two alternative approaches were discussed to determine the motor power according to the maximum speed or acceleration performance requirements. Obviously the two criteria for final speed and acceleration performance do not necessarily lead to equal power requirements and the greater of the two power values must be selected. Once the motor power Pm is obtained, the other required properties of the motor can be determined. The maximum speed requirements lead to definite power values (Equation (7.73)), whereas the acceleration performance produces implicit results and needs a further selection process.
There are two means of having Pmfs > Pma or Pmfs < Pma. In the former case when the acceleration performance for different base velocities (e.g. Figure 7.54) is constructed, in the upper curve a horizontal line with the value of Pmfs will intersect the curve and the base velocity and wheel torque (from the lower curve) can be obtained. The motor torque based on the power Pmfs, denoted by TmP, can be calculated from:
provided that the reduction gear ratio ng is available. The motor speed usually has a limiting value and this may be used to determine the value of ng:
where ωmax is the limiting speed of the motor and vmax is the maximum vehicle speed. The value of ng will have an influence not only on the motor speed but also on its torque. A low value of ng results in a small motor speed but a large motor torque.
The motor torque must additionally fulfil the requirement of a desired grading at low speeds, that is:
in which FG is the grading force at steady low speeds:
The value of the motor torque obtained from Equation (7.79) will not necessarily be consistent with the result of Equation (7.77). If TmP larger than TmG is obtained, then the base velocity already determined is correct and ωb is calculated from:
Otherwise, vb must be recalculated from:
and the motor properties will be Pmfs, TmG and ωb calculated from Equations (7.81) and (7.82). It should be noted that in this case the acceleration performance is better than initially specified. In other words, the time for the desired speed vd will be lower than the desired time td. The whole process is shown in Figure 7.60 a.
Figure 7.60 The two cases of (a) Pmfs > Pma and (b) Pmfs < Pma

Now if Pmfs < Pma is the case, this means that when the performance curves for acceleration are plotted (see Figure 7.60b), the power values in the upper curve are all greater than Pmfs. In order to choose the proper power for the motor in this case, again the gradeability should be considered. In the lower curve with the value of TmG the base speed is found and in the upper curve, the power Pma is obtained. Hence for this case, the motor properties are Pma, TmG and ωb calculated from Equation (7.81). It is also worth noting that in this case the maximum speed of the vehicle will be greater than that initially specified.
For quick reference, the sequence for determining the motor properties is summarized below:
a. Determine the motor power(s) to achieve the desired speed(s) on level and sloping roads and take the larger value as the candidate for the motor power Pm.
- Generate the acceleration results similar to Figure 7.59.
- Calculate the ng from Equation (7.78).
- If the value of motor power obtained in (a) lies in the upper curve of acceleration performance (Figure 7.60 a), (it means the power for the maximum speed of vehicle is greater than the power for acceleration), then the motor power is Pmfs and:
Calculate the motor torque from both Equations (7.75) and (7.77) (TmP and TmG).
If TmP > TmG, then the motor properties are TmP and its related vb value.
If TmP < TmG, then the motor properties are TmG and vb obtained from Equation (7.82).
b. If the motor power value obtained in (a) does not lie in the upper curve of acceleration performance (Figure 7.60b), (it means the power for the maximum speed of vehicle is lower than the power for acceleration), then the motor power is Pma and:
- Calculate the motor torque from Equation (7.79) (TmG).
- In the lower curve of the acceleration performance, at the TmG value obtain the base velocity and in the upper curve, the motor power Pma.
Size of the Engine
In a series HEV, the engine must be able to produce enough energy necessary for the sustainable motion of the vehicle without utilizing the battery energy. At a cruising speed of vc, the required motor output power to overcome the resistive forces can be determined using Equation (7.73). The engine power must also include the power loss in the driveline, therefore the total engine power for cruising, Pec is:
where and
are the efficiencies of generator, electric converter, motor and driveline respectively.
In a general driving situation that also involves acceleration aG of the vehicle, the term should also be included inside the brackets. Since large accelerations occur at low vehicle speeds vL, the aerodynamic term can be ignored and for such cases the engine power for accelerating, Pea is:
In order to compare these two types of engine power requirements, let us define an overall aerodynamic coefficient per mass of vehicle cpm:
(7.85)
Then, it is possible to calculate a specific engine power (power per mass) for different speeds and accelerations. Figure 7.61 is generated for a cpm of equal to 2.25 × 10−4, rolling resistance coefficient of 0.02 and an overall efficiency of 0.65, for both low speed and high speed ranges. In the low speed range, a flat road is considered and for the vehicle accelerations of 1, 2, 3, and 4 m/s2, the engine power requirements at different speeds up to 20 m/s are calculated. In the high speed range, for slopes of 0, 2.5, 5.0 and 7.5%, the variations of engine specific power at different vehicle speeds are determined.
Figure 7.61 The engine specific power rating

To supply power for a cruising speed of 120 km/h on a level road, the specific power is 24 W/kg, whereas for the same speed on a 2.5% slope it will be 37 W/kg (for a 1200 kg vehicle the engine power values are 28.8 and 44.4 kW respectively). The first choice will also provide the necessary power for cruising at 93, 72 and 58 km/h speeds on roads with 2.5, 5.0 and 7.5% slopes respectively. This power from the engine may only partly support the necessary power for the steady acceleration of the vehicle (accelerations of 4, 3, 2 and 1 m/s2 up to 12, 18, 25 and 45 km/h speeds).
As the high accelerations of the vehicle occur within the constant torque phase of the traction motor, the acceleration is almost constant in this phase. For a high acceleration performance from the standstill up to base speed vb (km/h), the variation of acceleration during the constant torque phase will be something like that shown in Figure 7.62. For a base speed of 50 km/h in 5 seconds, an acceleration of around 3 m/s2 is needed. This requires a motor power of around 60 kW (see Figure 7.59) and an engine power of about 90 kW. For this vehicle the 0–100 km/h time is around 12 seconds (see Figure 7.56). Thus even the second choice for the engine (44.4 kW) can only produce half of the required power for such acceleration. Increasing the engine size will increase the weight and cost and therefore it is better to have the remaining power supplied by the battery if such high accelerations are required.
Figure 7.62 Constant torque accelerations at different 0-vb (km/h) times

Size of the Battery
The battery must produce enough power to achieve the required performance over a multitude of driving conditions. In the previous section we discussed a design case in which the instantaneous power requested by the motor was greater than the supplied engine power. The deficit must be produced by the battery. In mathematical form, the battery output power is:
in which the first term is Pin the input power to the motor. The capacity of a battery is usually given in kW-hour (kWh). Determining the size of battery for the vehicle requires additional information including the ‘all-electric’ range of the vehicle and charging/discharging patterns. If the vehicle is designed to operate over large electric ranges (e.g. 60 km), obviously it will need larger capacities.
The charging/discharging pattern also has an important effect on the battery size. Let us rewrite Equation (7.45) in the form (assuming a constant working voltage):
(7.87)
in which E0 is the battery capacity in Wh. At time t1 SOC is:
(7.88)
Thus ΔSOC = SOC(t2) − SOC(t1) is:
(7.89)
The integral term is the net energy ΔE, taken from or given to the battery in the time span t1 to t2. For a zero ΔE the SOC will remain unchanged, whereas for negative and positive ΔE values, the SOC will increase or decrease respectively. If both the ΔSOC and ΔE can be estimated in a reasonable working interval, then the battery capacity E0 simply is:
The working range for SOC discussed earlier (see Section 7.4.3.3) is a good value for ΔSOC and ΔE is best determined over a wide range of battery operation in which the value of ΔSOC occurs. The vehicle starts with the battery's upper working limit SOCH and it must finish at the same SOC level at the end of driving cycle. Energy is supplied to the battery to keep its SOC unchanged between the start and the end of the cycle. The net energy consumption during such cycles simply is the average power consumption (efficiency of the battery included) times the cycle time Δt. Therefore the battery capacity can be determined from the following equation:
Example 7.6.2
Determine the component sizes of a series HEV with the vehicle properties of Table 7.11, in order to achieve the following performance:
a. maximum speed of 160 km/h on a flat road;
b. gradeability of 5% at 120 km/h;
c. 0–100 km/h acceleration time of 15 seconds;
d. gradeability of 45% at low speeds;
e. all-electric range of 20 km at speed of 60 km/h.
The maximum allowable motor speed is 6000 rpm. The maximum and minimum SOC values are 0.8 and 0.2 respectively.
The overall efficiencies and
are 0.85, 0.95, 0.85 and 0.95. The battery efficiency is 0.9.
Solution
Motor Properties
The sequence introduced for the motor sizing will be used. To obtain the first requirement of 160 km/h final speed, the motor output power simply is (Equation (7.70))
The second requirement similarly is: . The numerical result of 44.5 kW for this case is lower than that for the maximum speed. Thus Pm = 48 kW must be selected.
For (c) the MATLAB program of Example 7.6.1 can be used for td = 15 s, and the result is shown in Figure 7.63. The ratio of reduction gear is obtained from the motor speed limitation (Equation (7.78)): .
Figure 7.63 Acceleration performance results for Example 7.6.2
For the motor power of 48 kW, the base speed and wheel torque are 13.4 m/s and 1020 Nm. The corresponding motor torque then simply is TmP = 1020/4.24 = 240 Nm.
For (d) the tractive force must be larger than the resistive force at low speed and from Equation (7.79): TmG = 376 Nm.
Since TmP < TmG, then the motor torque must be taken TmG and vb from Equation (7.80) is: vb = 9.03 m/s and ωb = 1220 rpm. The motor properties are shown in Figure 7.64. With these values, the performances of the vehicle are shown in Figure 7.65. It is clear that the final speed and gradeability requirements are exactly met, while the final speed at 5% slope is improved (125 km/h instead of 120 km/h). Also the required time td is reduced to 13.5 second from initial 15 seconds (not shown in Figure 7.65).
Figure 7.64 The motor properties for Example 7.6.2
Engine Power
Figure 7.65 The final performances of the vehicle in Example 7.6.2
From Equation (7.83) the engine power at the cruising speed of 120 km/h on a level road and with overall efficiency of 0.6521 is 34.9 kW. For the same speed on a 5% grade the power is a much larger value of 65.0 kW. The former is also suitable for an average acceleration of 0.75 m/s2 at average speed of 20 m/s.
Battery Size
For a motor overall efficiency of 0.85, the minimum battery output power from Equation (7.86) is 21.7 kW.
To determine the battery capacity, the average power and its duration can be obtained from:
The battery capacity from Equation (7.91), therefore, is: E0 = 4.4 kWh.
7.6.2.2 Parallel HEV
The electric-only mode of operation of a parallel HEV depends only on its electric motor specifications, however, only a limited performance is usually expected in electric-only mode. For the desired performance of the vehicle in this mode, the electric motor can be designed based on the methods discussed earlier in previous section. In practice, the design of the electric motor, however, must be performed in relation to the design of the engine, as the two traction sources produce the motive force for the motion of the vehicle.
Engine Power
The engine in a parallel HEV is designed to propel the vehicle at high steady speeds without any help from the electric source. At a desired high speed vH the tractive power balances the resistive power and the motive power must also overcome the driveline losses. The necessary engine power, therefore, is:
(7.92)
The design could be analyzed for a flat road (θ = 0) or a mild slope at a desired speed vH. It should be noted that the high steady speed of the vehicle is not determined by the engine power, but is governed by the engine torque and the overall gear ratio (see Chapter 3). Therefore, the power rating obtained from this analysis is only an initial estimation of the engine maximum power and should be finalized when the torque-speed characteristics are also available.
Electric Motor Power
Here we consider a parallel hybrid with only one electric motor that is connected to the driving wheels through a single reduction gear. The electric motor is designed to supply assisting power for the high acceleration part of the motion as well as the low speed stop-start portions of the driving cycles. As the driving conditions are extremely varied, an exact estimation of the motor properties is impossible.
As an attempt to estimate the motor power, it is reasonable to consider the high acceleration motion of the vehicle in which a desired speed vd is expected during a specified time td when the vehicle starts from the rest. Such vehicle performance must be achieved by simultaneous supply of power from both the engine and electric motor. For a total power of Ptot supplied by both the engine and motor, the governing equation is (see Section):
in which:
(7.94)
(7.95)
(7.96)
(7.97)
(7.98)
(7.99)
(7.100)
For a given desired speed vd and time td, the total power can be determined by the application of Equation (7.93). Then, the motor power for high acceleration can be regarded as the difference between the total power and the engine power:
It is important to note that the acceleration performance is actually based on the availability of the total tractive force and not the power. Hence the assumption of a total maximum power delivery by motor and engine does not necessarily lead to an actual tractive effort with a desired acceleration performance.
The other requirement from the motor is to produce sufficient tractive power for low speed stop-start circumstances. This includes a variety of driving conditions with different power requirements. An estimate for this power can be obtained from well-known standard driving cycles. At low speed stop-start motions the required power for the motor is (see Equation (7.84)):
The first term is the power requirement for the acceleration and depends only on the driving pattern. The second term is the power requirement due to the resistive forces and depends mainly on the slope of the road. In order to evaluate the magnitudes of each part in Equation (7.102), we shall examine a standard urban driving cycle in the following example.
In a design process for the electric motor, different driving cycles and grading requirements can be considered at low speed stop-start motions so that the tractive force is only produced by the motor. The maximum value of motor power obtained in this process is Pmsg of Equation (7.102). It should be noted that Pmsg should not become unnecessarily large by assuming larger grades, because in such circumstances the engine torque can also assist the motor.
In any case, after the two power values Pma and Pmsg were determined from Equations (7.101) and (7.102), the larger of the two should be taken as the motor power. The other motor specifications such as the torque and base speed values must be determined by using additional information.
Example 7.6.3
The speed-time information in the first 200 second segment of ECE15 driving cycle (see Section 5.3) is given in Table 7.12. (In the actual driving cycle this segment repeats four times.)
Table 7.12 Cycle information for Example 7.6.3
a. Plot the driving cycle.
b. Determine and plot the acceleration of the cycle.
c. Determine and plot the power requirement to accelerate a unit mass of the vehicle.
d. Determine and plot the power requirement per unit mass of vehicle to overcome resistive forces. Consider a rolling resistance coefficient of 0.02 and grades of 0, 10, 20 and 30%.
e. For a vehicle of 1200 kg mass and driveline efficiency of 0.95, determine the motor power requirement for this cycle.
Solution
a. The first 200-second segment of ECE15 cycle is plotted in Figure 7.66.
b. The cycle acceleration can be obtained by following MATLAB commands:
Figure 7.66 First segment of the ECE15 driving cycle
Where t0 and v0 are two row vectors containing the time and speed values. The plot of acceleration is given in Figure 7.67.
c. The power requirement to accelerate 1 kg of the vehicle is speed times the acceleration (v.aG) and can be determined by a simple command ‘va=a.*v’. The result is plotted in Figure 7.68.
d. The resistive forces include the rolling resistance, gravity and aerodynamic forces. Since the cycle is low speed, the aerodynamic force is small and is ignored (Equation (7.102)). The power per unit mass is simply FR.v/m. The following MATLAB loop simply obtains the results for the four grades of 0, 10, 20 and 30%:
Figure 7.67 Acceleration profile for the driving cycle of Figure 7.66
Figure 7.68 Specific power due to acceleration for the driving cycle of Figure 7.66
Figure 7.69 illustrates the variation of the power requirement for the unit mass of the vehicle.
e. For the given vehicle, the maximum power requirement for acceleration on this cycle according to Figure 7.68 is 8.2×1200/0.95 or 10.4 kW. The power requirements to overcome the resistive forces according to Figure 7.69 are around 3.0, 20, 36.5 or 53 kW on a flat, 10%, 20% or 30% grade respectively. The total motor power is the summation of the two parts (Equation (7.102)).
Figure 7.69 Specific power due to resistive forces for the driving cycle of Figure 7.66
Size of the Battery
The battery must be capable of producing instantaneous power to achieve performance requirements as well as containing enough energy for the continuous operation of the system. The electric power of the battery in a parallel hybrid vehicle should be larger than the demand of the electric motor, or:
The battery capacity is designed in order that the motor energy requirements are met either during a pure electric acceleration phase or during motion on an urban driving cycle. Equations (7.90) and (7.91) are still applicable for this purpose.
Example 7.6.4
Determine the component sizes of a parallel HEV with the same properties of Example 7.6.2 and the same performances except:
- Consider a 0–100 km/h time of 10 seconds.
- The motor must be capable of propelling the vehicle on a 5% slope ECE15 driving cycle.
- Consider an all-electric range of 10 km on an ECE15 flat cycle with no regeneration.
- The minimum and maximum SOC values are 0.4 and 0.8 respectively.
- The overall motor and battery efficiencies are 0.85 and 0.9.
Solution
The design steps are straightforward, nevertheless to follow the solution more easily a MATLAB program is also provided in Figure 7.70 and its accompanying function in Figure 7.71.
Engine Power
As the requirement is the same as for the Example 7.6.2, the engine power rating in this case is again 48 kW.
Figure 7.71 The MATLAB function for Example 7.6.4
Motor Power
To obtain the motor power from Equation (7.101), an iteration process is involved. This has been performed in the function ‘Ptot’. Upon the return from the ‘fsolve’ statement the total power to obtain 0–100 speed within 10 seconds is 57.3 kW and Pma is 57.3 − 48 = 9.3 kW. However, by making use of Figures 7.68 and 7.69 (interpolate for 5% slope), Equation (7.102) results in Pmsg = 20.6 kW. Thus the motor power is selected as 21 kW.
Battery
From Equation (7.103) the power of the battery should be larger than 24.2 and, for instance, 25 kW could be selected.
For the battery capacity the requirement is 10 km of travel on an ECE15 driving cycle. The total power consumption during the cycle with no regeneration can be obtained by adding up the results of Figure 7.68 and the lower part of Figure 7.69 multiplied by the vehicle mass. The result for the positive part is illustrated in Figure 7.72. The average value of this power divided by the efficiencies of the motor and the battery is 2.4 kW. The total energy consumption during the 10 km travel as the value of average power times the total travel time is 1.32 kWh. The battery capacity from Equation (7.91) is 3.3 kWh.
Figure 7.72 The positive power demand in a single segment of the driving cycle
7.6.3 Optimum Sizing
Hybrid electric vehicles are designed around minimizing the fuel consumption, exhaust emissions and manufacturing costs without compromising HEV performance such as vehicle maximum speed, expected acceleration and gradeability. Sizing the HEV components for performance, discussed in the foregoing section, does not necessarily lead to the above objectives. A more successful HEV design requires optimal sizing of its key mechanical and electrical components in order not only to maintain or enhance driving performance but also to minimize the objective parameters such as the fuel consumption, emissions, costs and wear under various driving conditions that have to be taken into account.
The optimization concept illustrated in Figure 7.73 implies that there is a set of optimum parameter values for the vehicle (e.g. engine power Pe, motor power Pm, battery size Eb, etc.) such that if the vehicle is used in various driving conditions with desired performance qualities, the energy consumption and emissions of the vehicle as well as several other objective measures would be minimized.
Figure 7.73 Component optimization concept

In mathematical terms, this is an optimization problem of the following general form:Find a solution
(7.104)
that minimizes the objective function:
(7.105)
subject to the following constraints:
(7.106)
in which:
xi (i = 1, 2,..., n) are the n optimum values of HEV parameters,
fi (i = 1, 2,..., m) are the m objective values that should be minimized,
wi (i = 1, 2,..., m) are the weighting factors for balancing among the objective values and
gi (i = 1, 2,..., p) are the p constraint equations that must additionally be met.
Examples for xi are engine and motor powers Pe and Pm. Examples for fi are fuel consumption FC and cost C. Examples for gi are 0–100 km/h time of 10 seconds (i.e. ) and the maximum speed of 180 km/h (i.e.
).
In theory, the optimization result must be independent of the vehicle driving patterns. In other words, in any driving conditions, the HEV components should be optimum. The optimization processes, however, are unable to solve the problem independently of driving patterns and instead consider various driving cycles in order to broaden their usefulness. Therefore, the design of an HEV that considers only a few driving cycles is likely to be only partially optimal.
The efficiency of an HEV, in addition, depends on the management of its different energy elements. A successful component optimization process of an HEV, therefore, should also include power control parameters. This means prior to component sizing, the control strategies for the power management of the HEV system should also be developed. There are several approaches to the solution of this problem (e.g. see [3]) but they are all beyond the scope of this book.
The energy expenditure of a hybrid vehicle is crucial to the goal of achieving improved fuel efficiency compared with conventional vehicles. This needs more efficient use of energy sources such as chemical (fuel) or electric (battery) energies. The instantaneous flow of energy therefore must be monitored and controlled in a hybrid vehicle in order that the total energy expenditure is kept as low as possible. This requires a power management strategy as the rate of energy consumption is the power. Thus a control system is necessary for the hybrid vehicle in order to manage all the engine, motor-generator, battery and transmission elements at their best operating points. Each element also requires a control system to regulate its outputs according to the desired values at different conditions. For example, an engine management system (EMS) is responsible for monitoring and controlling the engine operation in diverse working conditions (see Section 2.7). When an input is specified (throttle), then the EMS will control the injection and ignition systems in order to optimize the engine performance.
A supervisory control system with the structure shown in Figure 7.74 takes the overall control of the hybrid vehicle and determines the best operating point of each component according to the driver's demands and information received from the vehicle, environment and component outputs. The commands issued by this controller are fed to the local controllers of all components as their inputs and these low level controllers are responsible for regulating the required outputs of each individual component.
Figure 7.74 HEV control hierarchy

7.7.1 Control Potential
The potential for the energy efficient motion of the hybrid vehicles stems from the availability of two energy sources with the possibility of using them under more efficient working conditions than would be possible if only one source is used. The combination of the two sources allows each source to be used one at a time or both together according to load requirements and hence provides opportunities for energy optimization.
7.7.1.1 Engine Shutdown
Engine idling is one of the everyday occurrences in driving situations such as warming up the engine, waiting for someone, stopping in queues and in traffic. There have been debates on the necessity of turning the engine off at idling as the fuel is wasted and the air is polluted unnecessarily. Engine shutdown at vehicle stops, therefore, is useful in reducing pollution, heat, noise and fuel consumption. On the other hand, when a conventional ICE vehicle is at rest, the engine still provides power for some of accessories such as A/C and alternator (if the battery needs charging). Moreover a vehicle with a stopped engine cannot drive off immediately. In addition, starting the engine needs energy and frequent engine start/stops have damaging effects on the starter and the battery. Therefore a question remains of how frequently the engine can be stopped and restarted but relative to the extra costs and energy used. The answer is not simple due to the diversity of vehicles, engines and working conditions, but nonetheless, figures as low as ‘10 seconds’ have been proposed for the minimum vehicle stop time that the engine shut down is beneficial.
However, hybrid designs allow the possibility of shutting down the engine with no concerns. In other words, when vehicle stops for a period of time, the engine can be turned off but the A/C will still work and vehicle can drive off immediately on electric power.
7.7.1.2 Economic Regions for ICE
The engine fuel efficiency was discussed in Chapter 5 and it was pointed out that the fuel consumption will be lower if the engine works in low BSFC regions of engine torque-speed map. In HEVs it is possible to arrange for engine to work in its more efficient map areas (Figure 7.75) by changing its load at a specified working condition.
Figure 7.75 More efficient areas in engine torque-speed map

7.7.1.3 Regeneration
The vehicle attains kinetic energy when it accelerates to a certain speed. This energy is wasted (transformed into heat) in normal vehicles as the vehicle comes to a stop. In hybrid vehicles there is the possibility of regaining a part of the kinetic energy (regeneration) by using the generator to develop braking torque. There are other influencing factors that can affect the efficiency of regenerative braking, as discussed next.
The Generator
The function of the generator is to apply resistive torque on the wheel that in turn causes the tyre to produce the braking force (see Section 3.10). The torque of a generator is dependent on its rotating speed and power as shown in Figure 7.27 (for a motor). It is evident that only at low speeds can the generator apply high torques and when the speed is high the torque is low (i.e. low braking force). In other words, the generator is unable to produce high braking forces at high speeds.
The Battery
The battery SOC or its charging current impose constraints on the regenerating energy. When the SOC is high, charging the battery might not be possible. The charging current of the battery will always be a major limiting factor in absorbing the energy during regenerative braking. In addition, the efficiency of the generator and the battery also reduces the effectiveness of the regenerative braking. The mechanical power Pm available at generator input during braking is:
in which a(t) is the instantaneous deceleration (a positive value) and PR is the power loss due to resistive forces (i.e. rolling resistance and aerodynamic forces). The available power for the absorption by the battery during the regenerative braking is:
where ηgb is the overall efficiency of the generator-battery system during charging. Assuming a constant voltage V with a maximum current I for the battery during regenerative charging, the maximum absorbable power by the battery is:
If the rate of input mechanical energy is larger than Pbm/ηgb, the battery only absorbs Pbm otherwise the value in Equation (7.108) will be absorbed. The efficiency of regenerative braking at each instant is defined as the ratio of absorbed power Pb to the potentially available mechanical power at the generator input:
The overall efficiency of the regenerative braking during the braking phase is the ratio of relevant energies:
(7.111)
At low speeds so that Pm < Pbm/ηgb Equation (7.110) simply reduces to ηr = ηgb, so is Equation 7.111 for constant values of the overall efficiency of the generator-battery system.
Example 7.7.1
A hybrid vehicle with 1000 kg mass is to stop within 100 m distance with an initial speed of 100 km/h. If the battery with a voltage of 200 V can be charged at a maximum current of 100 A, what percentage of vehicle kinetic energy will be absorbed by the battery? The overall efficiency of the generator-battery combination is 0.85. Ignore the power loss due to resistive forces.
Solution
The total energy of vehicle simply is 0.5 mv2 = 385.8 kJ (the energy of rotating masses is ignored). The deceleration and time of stop are (the resistive forces other than the brake force are ignored):
Pbm from Equation (7.109) is 20 kW. According to Equation (7.107), before time the input mechanical power is larger than the maximum absorbable power. The total absorbed energy by the battery therefore is:
That is only 33.2% of the total energy.
The Driving Pattern
The driving pattern affects the value of energy that is lost during braking. For example, driving in city traffic entails a cycle of low speed accelerations followed by decelerations. Highway driving, on the other hand, involves higher speeds producing higher energies that are also lost with braking. Nonetheless, the energy recovery ratio in a hybrid vehicle under urban driving conditions can be higher for frequent low speed acceleration/deceleration cycles. The reason is that the high energy rates involved in highway speeds cannot efficiently be recovered by the electric system.
Example 7.7.2
The vehicle of Example 7.7.1 with the same battery travels the NEDC cycle. Compare the maximum recoverable energies during the urban and highway parts of the cycle shown in Figure 7.76 by obtaining the regenerative efficiencies. Ignore the power loss of resistive forces.
Figure 7.76 NEDC cycle of Example 7.7.2
Solution
The ECE part of the cycle is four times repetition of a three-part cycle. In the first part braking takes place in 5 seconds from an initial speed of 15 km/h. Maximum braking power during this period is mav0 = 3.47 kW < 20/0.85 = 23.53 kW. Thus the recovered energy is
In the second part, braking is done from 32 km/h in 11 s. The maximum braking power is 7.18 kW < 23.53 kW and the recovered energy in this part is Eb12 = 33.58 kJ. In part three, initially braking reduces the speed from 55 km/h to 35 km/h within 8 s and then to a complete stop in 12s. In both phases the available power is less than the maximum absorbable value and thus the absorbed energy is Eb13=99.2 kJ. The total recovered energy during the ECE cycle therefore is EbECE = 4×Eb1 = 560.64 kJ.
For EUDC cycle braking first takes place at 70 km/h and during 8 s speed is reduced to 50. In this part the braking power is still lower than 23.53 kW and Eb21=78.7 kJ. The second braking phase starts at 120 km/h and within 34 seconds the vehicle comes to a complete stop. The maximum mechanical power reaches 32.68 kW > 23.53. Similar to Example 7.7.1, the time at which the braking power reduces to 23.53 kW is 9.52 s (at a speed of 86.4 km/h). The recovered energy is obtained as Eb22 = 435.2 kJ. The total regenerative power in this phase is EbEUDC = 513.9 kJ.
The available energy values for ECE and EUDC are EECE = 659.6 kJ and EEUDC = 648.2 kJ. Therefore the efficiencies of regenerative braking are:
ηr(ECE) = 85% and ηr(EUDC) = 79.3%.
It is worth noting that a higher efficiency in the second phase of EUDC cycle compared to the value obtained in Example 7.7.1 is due to a lower deceleration in EUDC.
The Driving Axle
In normal braking, the braking forces are applied on both front and rear axles, however, the generator (usually M/G) is typically only attached to the driving wheels on a single axle. Therefore the maximum possible energy absorption will depend on the braking capacity of that axle (either front or rear). On the other hand, the required braking torques in vehicles are larger than those that a generator can produce and thus electric braking alone is not sufficient and conventional mechanical brakes are also therefore required on an HEV.
Braking Stability
The best braking performance is obtained if the braking force on a vehicle is distributed according to the ideal braking curve shown in Figure 7.77 that prevents the rear wheels from locking up before the front wheels. However, the brake forces in the vehicles are usually applied on a linear basis (brake force line) that satisfies the rear wheel lock-up criterion up to large decelerations. Therefore the regenerative braking should also follow the same rules and take a balanced share of the total braking force. More details on the braking performance and regenerative braking can be found in [5] and [6].
Figure 7.77 Brake force distribution

7.7.2 Control
In order to improve the fuel economy and reduce emissions, the power management controller has to decide which operating mode of the hybrid vehicle to be used, and to determine the optimal split between the two power sources while meeting the driver demands and sustaining the battery state of charge. This is usually performed according to different methodologies and strategies.
7.7.2.1 Methodology
Power management methodologies can be classified into two categories, namely rule-based and mathematical model-based techniques. The first type employs control algorithms such as simple or Fuzzy logic rules for decision-making. These control types classify the operating conditions into several cases and use if-then rules to choose the best operating point among the possible cases. Rule-based algorithms do not use formal mathematical approaches and instead rely on the expert knowledge of the system behaviour and thus their performance depends on how they are tuned.
The mathematical model-based methodologies use optimization techniques based on the mathematical models of the system components and determine the best instantaneous split of power between the two sources in order to achieve the best performance with minimum fuel consumption and emission. These methodologies include static and dynamic methods. The former technique uses the steady-state component efficiency maps whereas the latter takes into account the dynamic nature of the system when performing the optimization and as a result under the transient conditions the dynamic approach leads to more accurate results albeit at the expense of more intense computations.
7.7.2.2 Strategy
The overall strategy of power management control is effectively the set of rules that must be employed in order that the objectives of the control system are met. In the rule-based methodologies, the rules are directly applied in every instant and control outputs are determined. In other methodologies in which an optimization process is involved, an objective function is typically introduced and its magnitude is minimized while additional constraints are fulfilled (similar to the method discussed in Section 7.6.3).
The basic rules for the rule-based methodologies are based on the vehicle torque and power demands (see Figure 7.78):
- When the torque demand is higher than the engine torque, the motor should assist if battery SOC allows.
- At low speeds if power demand is low, only the electric motor is used since the engine is inefficient in such cases.
- At greater power demands below the motor maximum power curve, the motor alone can work if the SOC is large enough, otherwise the engine alone can deliver the power. A hybrid mode with both the motor and engine power split can also be used based on the efficiency of the system.
The basic strategy used in practice is charge sustaining which is beneficial in utilizing the maximum potential of electric propulsion. This means that whenever possible, the electric motor is used to propel the vehicle either in electric-only or in electric assist modes. This strategy is especially useful under stop-start driving conditions in city traffic in which the motor must launch the vehicle frequently. Obviously if the amount of charge of the battery or the SOC is to remain unchanged, the input and output energies to the battery must be balanced. The engine on/off technique is employed in order to supply energy to the battery when its SOC drops to a permissible low level. When no input power from the engine to the battery is needed (the SOC reaches its high level) and the vehicle propulsion is independent of engine, it can be turned off.
Figure 7.78 Tractive force-speed diagram with high torque and low power areas
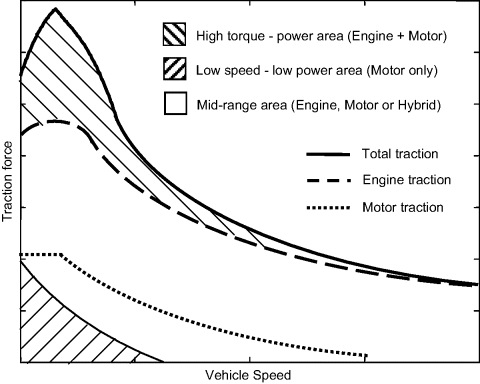
This chapter dealt with the fundamentals of hybrid electric vehicle concepts as well as design issues. The classification and operating modes of HEVs were discussed starting from the basic series and parallel types up to the more sophisticated power split versions. The characteristics of major HEV components and their modelling issues were explained. Then the performance analysis of the hybrid vehicles was considered and mathematical models were provided. Component sizing as an important issue in HEV design was considered and details of sizing based on vehicle performance were discussed. The HEV power management was briefly introduced and some overall design guidelines were discussed.
It is important to note that the crucial concept of hybrid vehicles is their energy efficiency compared to conventional vehicles. This is achieved by very careful design and use of the vehicle components at their most efficient points. Success depends on how the vehicle energy consumption is managed and how vehicle energy is regained through regenerative braking. The design of hybrid systems as well as their accurate performance evaluation requires specialist software as well as the availability of detailed component behaviours, in particular, their operating efficiencies.
1. What are the main reasons for the development of hybrid vehicles?
2. What is the basic classification for the hybrid electric vehicles?
3. In your view, is a series HEV more efficient than a conventional vehicle? Why?
4. Name and explain the operation modes of an HEV.
5. What are the benefits of the parallel HEV over the series-parallel HEV?
6. What is the main difference between the series-parallel HEVs and power-split HEVs?
7. Explain the degree of hybridization and the classification of hybrids based on it.
8. What are the power split devices?
9. Describe the power circulation? Is it useful?
10. What is the SOC and why is important?
11. Explain the BMS and its important tasks.
12. Describe the methods of sizing the HEV components.
13. Explain how the optimum sizing of an HEV component is related to the driving patterns.
14. What is the supervisory control in HEVs? What are the low level controllers?
15. Explain the potential areas available for an HEV controller in order to enhance the fuel economy and reduce the emissions.
16. What is regeneration? Explain the influencing factors in regeneration.
17. Explain the methodologies and strategies of HEV control systems.
Problem 7.1
Consider the simple PSD of Section 7.3.1 under a transient (dynamic) working condition and derive equations to replace Equations 7.4, 7.5 and 7.7.
Problem 7.2
Repeat Example 7.3.4 considering global component efficiencies of 0.8, 0.8, 0.95 and 0.98 for MG1, MG2, PSD and the reduction gear respectively. For MGs use identical efficiencies for motor or generator modes (Hint: Derive necessary relations for power losses and circulating power).
Problem 7.3
The vehicle of Example 7.3.4 starts the motion in ‘electric-only’ mode up to speed of 30 km/h, then the engine is started and the vehicle is propelled in ‘engine-only’ mode with full throttle. Plot the vehicle performance curves up to 20 seconds after motion starts. The motor has a base speed of 1200 rpm.
Problem 7.4
Work Example 7.3.4 for the EM-PSD with kp = 1.5
Problem 7.5
The overall efficiency of a battery can be regarded as:
in which Ed and Ec are the discharged and charged energies of the battery. A simple experiment is designed in which a battery cell is discharged and charged again in a controlled manner. Energy is given to a consumer from a battery at a constant current I during time tf. At this time period, the voltage of battery remains a constant V. Then the battery is charged at the same voltage V, current I and time tf:
a. Derive an equation for the overall efficiency of the battery.
b. For a battery with open terminal voltage of 11.2 V and internal resistance of 0.05 ohm, plot the efficiency of battery for different currents.
Problem 7.6
In Example 7.4.3 if the battery must be charged when the SOC is below 60%, determine the time at which the engine must be started to recharge the battery and also calculate the distance travelled by then.
Problem 7.7
Consider the vehicle and driving cycle of Example 7.4.3 and determine:
a. Total tractive energy consumption in a cycle;
b. Total regenerative energy and its ratio to the tractive energy.
Problem 7.8
Rework Example 7.5.1 by including the torque-speed characteristics of the motor that has a base speed of 1000 rpm but with the same maximum power.
Problem 7.9
Repeat Example 7.7.1 for maximum battery charging currents of 20, 50 and 70 A. Also plot the variation of regenerating efficiency with maximum charging current.
Results: 7.3%, 78.6% and 31.6%.
Problem 7.10
Repeat Example 7.7.2 for maximum battery charging currents of 20, 50 and 70 A. (Hint: write a MATLAB program.)
Result for I = 20 A: 78.6% and 31.6%.
Problem 7.11
Repeat Example 7.7.1 with a rolling resistance coefficient of 0.015 and overall drag factor of 0.3.
Problem 7.12
Repeat Example 7.7.2 with a rolling resistance coefficient of 0.015 and overall drag factor of 0.3.
Problem 7.13
The generator of Example 7.7.1 has a maximum torque of 200 Nm up to a base speed of 1200 rpm, and a reduction gear ratio of 5.0. The rolling radius of the wheel is 30 cm. Calculate the regenerative braking efficiency (The braking force is produced only by the generator).
Problem 7.14
Use the generator of Problem 7.13 with a maximum torque of 150 Nm to rework Example 7.7.2.
Alongside the upsurge of interest in hybrid vehicles since 2000, several textbooks have appeared on the subject dealing with electric vehicles (EVs), hybrid electric vehicles (HEVs) and fuel cell vehicles (FCVs).
An excellent introduction to EVs is provided by Larminie and Lowry [6]. The book is mainly descriptive and focuses on the design of EVs, with chapters specializing in their components – batteries and motors, for example. There is also some basic material describing fuel cells and the use of hydrogen as a vehicle power source. The book has been extensively used by undergraduate students and the Appendices include some simple, but useful examples using MATLAB code. The definitive reference on EVs is by Chan and Chau [7]. Professor Chan is a pioneer of EV technology in academic circles and has built up a world-recognized centre of excellence at Hong Kong University. The book concentrates on a comprehensive analysis of the design of EVs, but also includes discussion of more general issues relating to energy and the environment. Also, a substantial chapter is devoted to the problems of infrastructure and charging.
There are two good textbooks on HEVs. The textbook by Ehsani et al. [5] covers the fundamentals, theory and design of EVs, HEVs and FCVs. As well as providing the background theory and equations, design examples with simulation results are provided. The book stems from the research and teaching carried out at Texas A & M University and so it provides an excellent introduction to the subject area. The authors spend a considerable time describing the behaviour of internal combustion engines (ICEs) which of course, are just as important as the electrical components in a hybrid powertrain – and the ICE power map characteristics for hybrid applications are usually different from those designed for conventional vehicles. Miller's book [8] also provides an excellent reference book for EVs and HEVs. In particular, the benefits of different HEV architectures are critically compared; then in Chapters 3 and 4, Miller goes into admirable depth on the design specifications of hybrid powerplants followed by a detailed study of sizing the drive system. The entire book, therefore, has a strong practical design theme running through it.
Finally, for those readers who require further background information on fuel cells, there is another excellent introductory text by Larminie and Dicks [9] which provides a thorough overview of their design and performance.
[1] Ren, Q., Crolla, D.A. and Wheatley, A. (2007) Power Split Transmissions for Hybrid Electric Vehicles, in Proc EAEC 2007 Congress, Budapest.
[2] Mashadi, B. and Emadi, M. (2010) Dual Mode Power Split Transmission for Hybrid Electric Vehicles, IEEE Transactions on Vehicular Technology, 59(7).
[3] Guzzella, L. and Sciarretta, A. (2005) Vehicle Propulsion Systems: Introduction to Modelling and Optimisation. Springer, ISBN 978-3-549-25195.
[4] Wong, J.Y. (2001) Theory of Ground Vehicles, 3rd edn, John Wiley & Sons, Inc., ISBN 0-470-17038-7.
[5] Ehsani, M., Gao, Y., Gay, S.E. and Emadi, A. (2005) Modern Electric, Hybrid Electric and Fuel Cell Vehicles: Fundamentals, Theory and Design. CRC Press, ISBN 0-8493-3154-4.
[6] Larminie, J. and Lowry, J. (2003) Electric Vehicle Technology Explained. John Wiley & Sons, Ltd, ISBN 0-470-85163-5.
[7] Chan, C.C. and Chau, K.T. (2001) Modern Electric Vehicle Technology. Oxford University Press, ISBN 0-19-850416-0.
[8] Miller, J.M. (2004) Propulsion Systems for Hybrid Vehicles. Institution of Electrical Engineers, ISBN 0-86341-336-6.
[9] Larminie, J. and Dicks, A. (2003) Fuel Cell Systems Explained, 2nd edn. John Wiley & Sons, Ltd, ISBN 978-0-470-84857-9.