7
Quantification of Technical, Economic, Environmental and Social Benefits of Microgrid Operation
7.1 Introduction and Overview of Potential Microgrid Benefits
Depending on its operation strategy (see Figure 1.5), a microgrid can provide a large variety of economic, technical, environmental, and social benefits to both internal and external stakeholders. This chapter analyzes such benefits in detail, based on typical networks identified for a number of representative EU member states. The study has been completed in the framework of the EU R&D project, More Microgrids [1].
Figure 7.1 provides an overview of microgrid benefits in economic, technical and environmental aspects. Each benefit item is mapped to the related stakeholder with dotted lines. Obviously, identification of microgrid benefits is a multi-objective and multi-stakeholder interest coordination task.
Figure 7.1 Overview of microgrid benefits
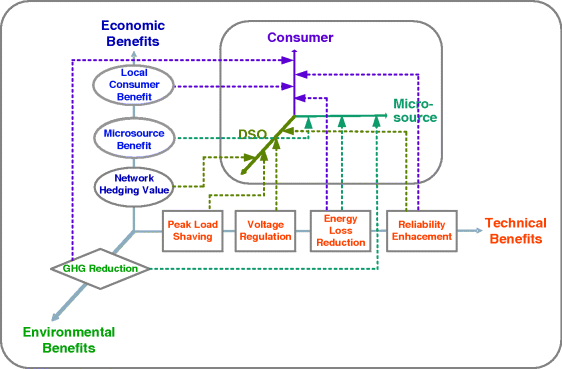
Before going into details of a quantitative analysis, a brief qualitative overview of these benefits is given.
7.1.1 Overview of Economic Benefits of a Microgrid
The economic values created by a microgrid can be roughly categorized into providing locality benefit and selectivity benefit. Locality benefit is mainly attributed to the creation of an internal “over-the-grid” energy market within the microgrid, where microsource units could sell at prices higher than the prices at wholesale level and end consumers could buy at prices lower than the retail level. This is equivalent to Market Policy 1 (good citizen behavior), analytically described in Section 2.7.2. Selectivity benefit is associated with optimization of real-time dispatch decisions that minimize opportunity cost of the whole microgrid with consideration of technical and environmental constraints. This is equivalent to Market Policy 2 (ideal citizen behavior), analytically described in Section 2.7.2.
Both locality and selectivity benefits can be attributed to either the consumer side or the microsource side, where a proper market design/interest allocation mechanism is needed to ensure a reasonable split of both benefits between consumer and microsource owner. From a macro-economics point of view, the locality benefits of a microgrid can be grouped into two aspects:
- microgrid can act as an initiator of local retail and service markets,
- microgrid can act as a hedging tool against potential risks of price volatility, outage, load growth and so on.
The selectivity benefit of a microgrid can also be further categorized in the following two aspects:
- microgrid can act as an aggregator of both supply- and demand-side players,
- microgrid can serve as an interest arbitrator for different stakeholders.
7.1.2 Overview of Technical Benefits of a Microgrid
A microgrid can potentially improve the technical performance of the local distribution grid mainly in the following aspects:
- energy loss reduction due to decreased line power flows,
- improved voltage quality via coordinated reactive power control and constrained active power dispatch,
- relief of congested networks and devices, for example during peak loading through selective scheduling of microsource outputs,
- enhancement of supply reliability via partial or complete islanding during loss of main grid. When the total number of microgrids reaches a sufficiently high share in LV substations, similar technical benefits can be expected in upstream grids as a consequence of multi-microgrid operation.
The actual level of technical benefits depends mainly on two factors: the optimality of microsource allocation and the degree of coordination among different players. Effective planning of microsource size and location can maximize unit contribution to system performance and random interconnection of oversized microsources in weak grids, but it can create more technical problems than the benefits it provides. It is also critically important that a real-time, multi-unit coordination platform, in either centralized or decentralized form (see Chapter 2) is needed to maintain targeted microgrid technical performance at all times.
7.1.3 Overview of Environmental and Social Benefits of a Microgrid
Environmental benefits of a microgrid can be expected from two aspects: shift toward renewable or low-emission (e.g. natural gas) fuels and adoption of more energy-efficient energy supply solutions (e.g. combined heat and power applications) including demand-side integration. With widespread national support policies for distributed renewable resources – for example, photovoltaics – the fuel-switching credit of microgrid is expected to grow as RES costs decrease over the years. Application of CHP and district heating and/or cooling concepts, on the other hand, varies significantly from region to region and is expected to find considerably different levels of acceptance across Europe. Demand side response, either manual or automated, is also greatly facilitated by the controlled operation of a microgrid.
Social benefits of microgrids can be mainly expected from:
- raising public awareness and fostering incentives for energy saving and GHG emission reduction,
- creation of new research and job opportunities,
- electrification of remote or underdeveloped areas.
All of these listed impacts, however, can be seen as long-term effects, and although qualitatively easy to understand, can be very difficult to quantify.
7.2 Setup of Benefit Quantification Study
7.2.1 Methodology for Simulation and Analysis
For this book, an analysis of different microgrid benefits was carried out on a yearly basis using the sequential Monte Carlo simulation method (Figure 7.2). Due to the cyclic nature of both electricity market prices and renewable (e.g. photovoltaic) output, intraday microgrid schedules are created and evaluated as hourly settlement results. Annual outcome of the optimization algorithm is consequently a statistical summary of individual daily simulations. In this section, the general framework of this analysis is outlined.
Figure 7.2 Algorithm overview of microgrid benefit quantification study
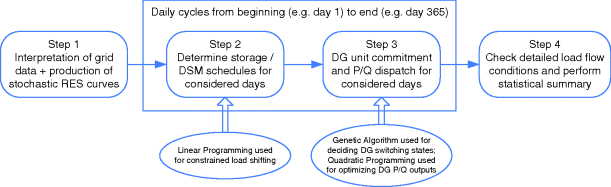
Typical annual profiles of load, RES generation and market prices have been synthesized, based on measurements or historical data. As microgrids are typically located at LV level, with mainly small residential or commercial customers, there are significant variations in load curves, which, in combination with the uncertainties from RES output as well as (wholesale) electricity price, turn the day-ahead microgrid scheduling task into a highly stochastic problem. Since prediction errors are inevitable, potential microgrid benefits evaluated from simulated or forecast data should be viewed as best-case results – that is, actual operation decisions might be sub-optimal, thus leading to lower benefits.
In order to deal with the modeling requirements of different microsource types, RES units, storage and demand-side response resources are dispatched at priority, and then the DG unit schedules are created for active and reactive powers, respectively. For the DG unit commitment, genetic algorithms or simply priority lists can be used to determine the optimal DG on/off states. Quadratic/linear programming models can be used for optimal power dispatch, as discussed in Section 2.7.4.
The formulation described in Section 2.7.4 can be further expanded by assigning cost functions to greenhouse gas (GHG) emissions and technical aspects, such as losses or reliability of supply and external factors can be internalized within the economic criteria, leading to an economic formulation of the optimal control strategy problem that considers:
- retail revenues collected from end customers,
- revenues of selling electricity to wholesale market,
- total cost of buying electricity from wholesale market,
- overall generation cost of DG (fuel, startup/shutdown, operation and maintenance cost),
- total emission cost from electricity generation,
- cost of energy losses in the grid,
- total cost of electricity generated from RES units,
- total control and communication costs for operating the microgrid.
Obviously, this total opportunity cost objective function is an artificial entry in a liberalized electricity market, as it represents the interests of multiple entities, and, in practice, requires extensive collaboration and compromise among the various actors.
For the optimization problem, three sets of system operational constraints are applied:
After creation of a complete schedule, load flows are run to calculate power losses and to check voltage and loading conditions in the network. Due to the large amount of output data, network variables are recorded as discrete probability density functions via a statistical summary procedure.
7.2.2 European Study Case Microgrids
It is not possible to quantify microgrid benefits considering a “typical” average system. Constitution and performance of microgrids are generally subject to a huge variety of internal and external factors, which can be summarized under the following three categories:
To quantify microgrid benefits at European level, an evaluation framework was developed to accommodate the majority of input and output parameters at a manageable level of data complexity. Based on this framework, typical European distribution networks of different types were studied, as shown in Figure 7.3. The results of this study are presented in Section 7.3. These results are critically influenced by the assumptions listed in the following subsections of Section 7.2, and therefore should be treated only as indications of future trends and the type of studies required.
Figure 7.3 European study case networks (DE = Germany, DK = Denmark, GR = Greece, IT = Italy, MK = FYROM, NL = The Netherlands, PL = Poland, PT = Portugal, UK = United Kingdom)
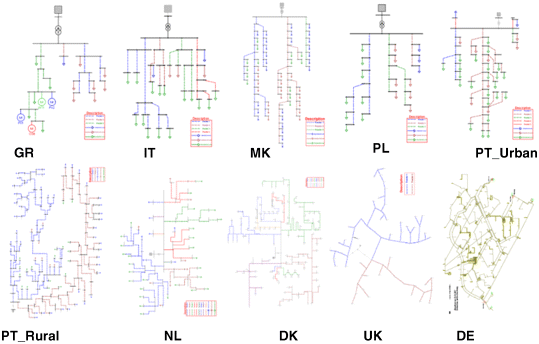
Installed generation capacity and annual energy consumption vary significantly across the different European countries examined. Figure 7.4 demonstrates the basic assumptions made in the study: average wholesale prices are 40–90 /MWh, national CO2 emission levels vary between 400 kg and 800 kg/MWh and end user retail electricity prices (split into energy, network charges and taxes) are 6–28
ct/kWh.
Figure 7.4 Retail/wholesale tariff structures and emission levels considered

7.2.2.1 Development of European Microgrids from 2010 to 2040
For the adopted evaluation framework, four future microgrid scenarios are defined for the years 2010, 2020, 2030 and 2040, that satisfy 10, 20, 30 and 40%, respectively, of their own demands by RES and bio-fueled heat-driven CHP units. This facilitates cross-national comparison, despite the regional differences in terms of adopted microsource technologies. In order to minimize the potential number of simulation cases, microsource allocation is assumed to be optimal for all cases. The RES and CHP self-supply level of the microgrids annual energy needs is kept below 50%. This is essential, since a higher level of self-supplied energy ratio would imply that the RES power installations (i.e. penetration level in terms of power rating) should be higher than 150% in countries where microgrids are dominated by intermittent RES technologies with lowfull load hours (FLH) (< 2000 h/a). The FLH is defined as the equivalent number of hours that DGs would operate under nominal capacity, in order to provide the same amount of annual energy. As active power outputs from intermittent RES and heat-driven CHP units are not naturally dispatchable, the controllability of a microgrid relies mainly on the installation of dispatchable microsources and storage devices.
After definition of a microgrid configuration aimed at satisfying local energy needs, the share of typical microgrids within national power systems (microgrid dissemination ratio) per country and region are assumed to be as shown in Figure 7.5, for 2010, 2020, 2030 and 2040, with pessimistic (P) and optimistic (O) assumptions.
Figure 7.5 Microgrid dissemination ratio in EU national grids (O = optimistic assumptions, P = pessimistic assumptions)
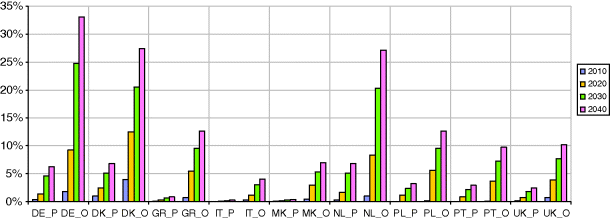
Detailed penetration scenarios of RES and CHP units assumed in each simulated case are given in Figure 7.6. It is noted that countries with high RES energy production from PV (IT, PT) or wind turbines (PL) are likely to have microgrid configurations of more than 100% RES penetration levels (installed microsource capacity compared to maximum load) by 2040.
Figure 7.6 Typical microgrid configuration per country (U = urban networks, R = rural networks)
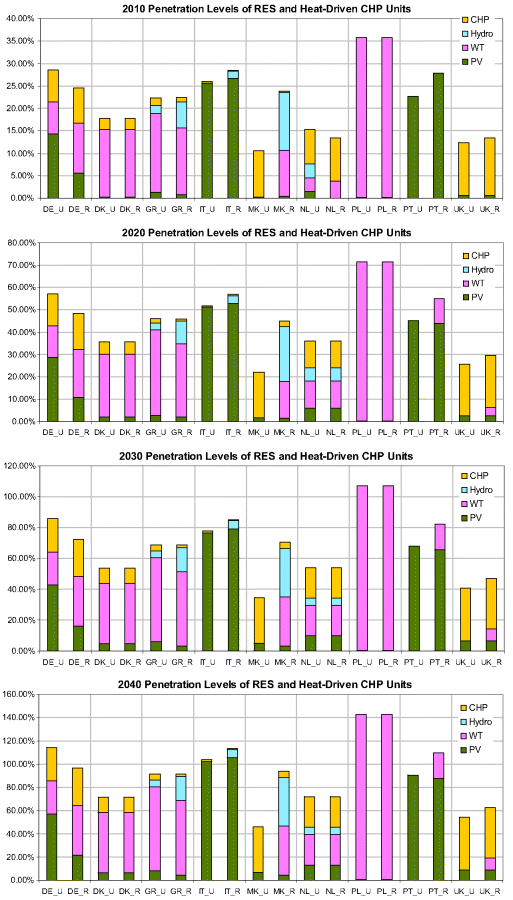
7.2.2.2 Basic Cost Assumptions
Figure 7.7 shows the range of cost/kWh microsource generation, assumed for different technologies between 2010 and 2040. High cost reductions are expected for PV and fuel cell technologies from 2010 to 2040, while the costs of other RES technologies are assumed to be more stable over the years. Natural gas based microsources are assumed to face increasing fuel costs in the future.
Figure 7.7 Assumptions of microsource generation costs
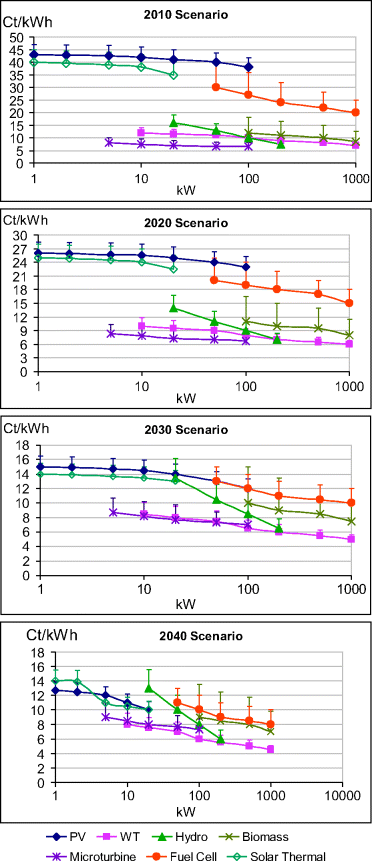
For a consistent calculation of net present value (NPV), interest rate (depreciation rate) of all examined countries is assumed to be constant at 5% throughout the examined period. Electricity market price levels are assumed to remain constant over time.
Dispatchable micro-generators are the main controllable generating units within a microgrid, so system operation optimization decisions will be strongly dependent on their cost functions, which can be expressed as:
(7.1)
Costs for control, metering and communication mainly depend on the complexity of the microgrid (the number of nodes to be monitored and controlled). Assuming 5000 h/a as FLH of load demand, the average annual energy demand observed per node (as load substation) is 50–160 MWh/a for most microgrids tested.
Ensuing cost calculations for control, metering and communication can be described as follows:
It should be noted that the differential cost of transforming a distribution network with DER to a microgrid comprises exactly the costs for control, metering and communication. Equation (7.2) suggests that microgrid benefits must exceed 0.6–4 /MWh to justify active control measures. As this additional cost is not dependent on microsource operation decisions, it is used for comparison with the estimated microgrid benefits.
7.3 Quantification of Microgrids Benefits under Standard Test Conditions
7.3.1 Definition of Standard Test Conditions
Due to the comparatively large number of different assumptions that could impact microgrid benefits, a basic standard test condition (STC) is defined to evaluate microgrid benefits under a “most likely to happen” scenario. Mid-level wholesale market price levels corresponding to 80% of the peak in the past decade are assumed per country (Figure 7.4). Real-time and directional price setting is applied, and a combined operation mode – as defined in Section 1.5.3 – is assumed. Sensitivity analysis with regard to these preset conditions is performed in this section to evaluate microgrid performance under various evaluation indices.
7.3.2 Balancing and Energy Results
Figure 7.8 demonstrates the percentage of local load supplied by microsources and the share of microsource generation in the supply of local loads for different countries and different microgrid setups under STC. The load-side self-supply level is the percentage of microsource-supplied load demand over the total annual load. The microsource-side self-supply level is the ratio of microsource-generated electricity that is consumed by local load as a percentage of their total generation.
Figure 7.8 Microgrid self-supply level on demand and supply side (U = Urban networks, R = Rural networks)
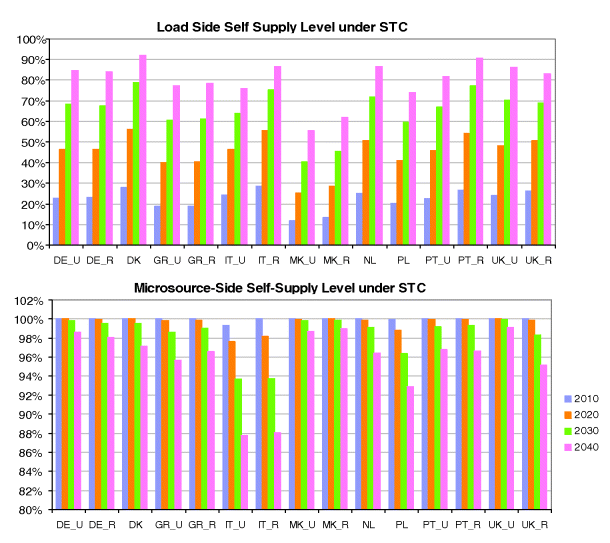
Most microgrids start with a load-side self-supply level of 15–25% in 2010 and reach 70–90% of local demand by 2040. FYROM is the only case where the self-sufficiency level starts from 10% in 2010 and ends up with around 60% in 2040, mainly due to lower wholesale and retail prices, leading to low microsource utilization. Even with high microsource penetration levels (up to the case of 2040), the differences between retail and wholesale prices lead microsource units to supply local loads most of the time. A general local supply ratio of >95% can be observed with the exception of Italy, where high electricity prices tend to encourage microsource units to export more often than the average level seen in the other countries.
Figure 7.9 illustrates the full load hours (FLH) of dispatchable microsource units under STC. It is noted that countries with high initial FLH values in 2010 (>6000 h/a) generally experience a reduction of FLH as time progresses to 2040. Countries starting with low FLH value (i.e. Greece and FYROM) show increasing FLH values as time passes. The very low FLH hours (<3000 h/a) of FYROM fully explains its low self-sufficiency level shown in Figure 7.8.
Figure 7.9 Full load hours of dispatchable microsource units under the standard test conditions
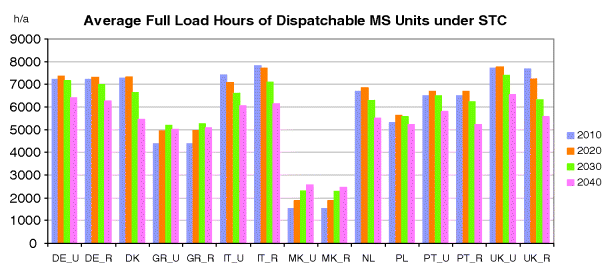
Figure 7.10 shows the estimates of the average per-kWh premium support levels (on top of microgrid earnings) in order to ensure the profitability of subsidized RES units. The high initial values noticed in Italy and Portugal can be mainly explained by the high penetration levels of expensive technologies (PVs) assumed for both countries. It is shown that in the majority of countries, financial supports for RES units within a microgrid by 2030 or 2040 will largely not be needed, as the internal trading prices are already sufficient for ensuring general unit profitability without market distortion. Slower adaptation of RES units to the fully commercialized microgrid internal market can be explained either by the high costs of the assumed microsource technology (e.g. PV for Portugal) or by the low electricity prices (e.g. in FYROM).
Figure 7.10 Premium support level for RES units under STC
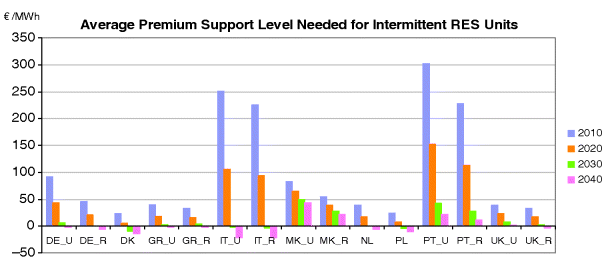
7.3.3 Technical Benefits
7.3.3.1 Reduction of Losses and Voltage Variations
DER can provide a number of technical benefits in the operation of the distribution network, even if they are not actively controlled (passive DER operation). However, the coordination platform provided by microgrids enables the extension of these benefits to more sections of the grid, at more critical times and for serving simultaneously multiple purposes. Therefore, the technical benefits of a microgrid result from the coordinated control of all installed DER units. This probably calls for more complicated reward schemes for the DER owners.
Figure 7.11 shows the loss reduction and the voltage variation reduction in the LV network within a microgrid. Reduction of system losses (DSO perspective) refers to the reduction of annual energy losses under microgrid operation compared to the passive DER operation, that is:
Figure 7.11 Annual energy loss reduction and voltage regulation credit
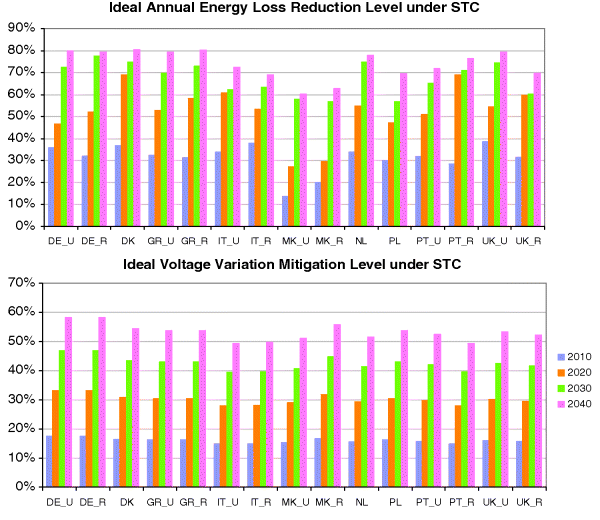
Reduction of system loss (%) = 1 − (annual energy losses under microgrid)/(annual energy losses under passive grid operation)
Reduction of voltage variation refers to reduced maximum absolute voltage deviation at worst voltage quality node(s) under microgrid operation compared to the passive DER operation, that is:
Reduction of voltage variation (%) = 1 − (max absolute voltage variation in microgrid)/(max absolute voltage variation under passive grid operation)
Figure 7.11 corresponds to the ideal situation of optimal allocation of microsources. A good correlation of loss reduction to (load-side) self-sufficiency level. Figure 7.8 shows that microgrids with optimally located microsources present significantly lower energy losses, since the majority of load demand is met locally by the microsource units. In practice, however, the DSO or microgrid operator has little or no control over the size and location of the DER. This means that the calculated loss reductions may have only a theoretical reference value. In fact, in some extreme cases, interconnection of disproportionally large microsource units at weakly loaded networks might increase energy losses. Therefore, it is very important that the microgrid planner or regulator provides the proper incentives, that could ensure efficient and effective microsource interconnection schemes to maximize the technical benefits from the microgrid operation. This also applies to the maximization of voltage and loading improvement benefits.
It should be noted that high R/X ratios of distribution lines result in the close coupling of active power with voltage magnitudes, as analyzed in Chapter 3. Load flow simulations of the studied networks have shown that the reactive power control of the microsource units could only contribute approximately 10–30% of the total voltage regulation. Since the active power output of the intermittent RES units is assumed non-controllable, the voltage is controlled primarily by the dispatchable microsources. This is the main reason why the effects on microgrid voltage control are less prominent.
7.3.3.2 Peak Load Reduction
Reduction of peak loading (DSO perspective) refers to the reduction of peak loading in the worst-loaded branch under microgrid operation compared to passive grid operation, that is
Similar to voltage regulation, peak reduction depends heavily on the location and the installed capacity of dispatchable microsources (Figure 7.12). The effect of reactive power control on line currents seems to be even smaller than its effect on voltage regulation.
Figure 7.12 Ideal peak load reduction credit, STC
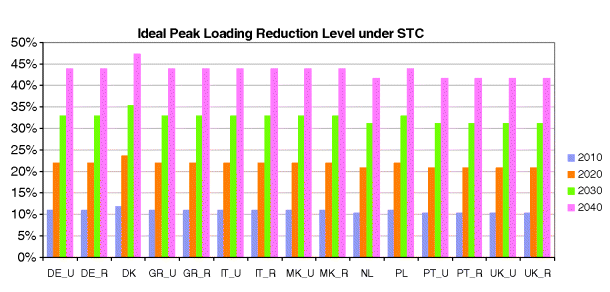
7.3.4 Economic Benefits
7.3.4.1 Economic Benefits of Consumers
Local consumer benefit (end consumer perspective) refers to the theoretical room for reduction of retail prices. It is based on the assumption that all economic benefits of a microgrid will be enjoyed by the end consumers, which is most likely to happen under the prosumer consortium ownership model, described in Section 1.5.2, where consumers own and operate multiple microsource units as an aggregated prosumer entity. The benefit can be defined in either relative (per unit) or absolute terms (/kWh) as follows:

Figure 7.13 shows the maximum total economic benefit of the consumers side in both absolute and relative terms. The economic benefits are the sum of the potential economic benefit obtained from the possibility to choose from more energy suppliers due to market operation, which is termed as market selectivity value, and due to RES proximity to the consumer side, which is termed as locality value. These benefits are calculated under standard test conditions.
Figure 7.13 Maximum total consumer benefit
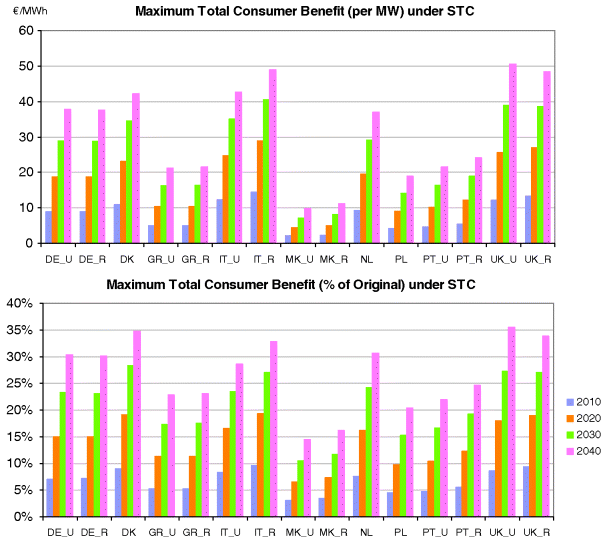
The consumer-side selectivity benefits are based on the assumption that all unsubsidized microsource units are operated under zero profit (e.g. when end consumers own the microsource units) and the DSO does not impose any distribution network usage charge for the energy consumption within the microgrid. Comparatively large variations of load-side selectivity benefits have been observed in the various cases due to the different FLH values of dispatchable microsource units and the profit margins between retail prices and microsource costs. As essentially FLH of microsource units is also determined by market prices, the consumer-side selectivity benefit level can be seen to be extremely sensitive to electricity market prices.
Unlike benefits obtained from market selectivity, RES locality benefit is totally dependent on their financial support. This means by default, that market forces will not provide such benefits to consumers due to natural cash flows within a microgrid. If a microgrid comprises only subsidized RES units that are priority dispatched, then consumers will not enjoy any selectivity benefit and the RES locality value – avoided use of system (UoS) charge – will be the only benefit for them. As consumer-side RES locality benefits are deduced from the standard grid charges, UoS charge tariffs determine the national variations in locality benefit value.
Overall, cost saving potentials range from 0.002 Ct/kWh in 2010 to 0.05 Ct/kWh in 2040. The majority of results indicate cost savings ranging from 7% ± 5% in 2010 to 25% ± 10% in 2040. These savings do not include taxes incurred in retail prices that vary widely among the examined countries.
It should be noted that consumers will enjoy the above benefits only with market policy 1 (Section 2.7.3), while with market policy 2, they will have to pay the same electricity prices as without microgrids.
7.3.4.2 Economic Benefits of Microsource Owners
Microsource benefit (microsource perspective) mainly refers to the maximum potential of increasing the selling price under a microgrid setting. This benefit is of course based on the assumption that consumers and the microgrid operator do not benefit economically from the microgrid operation. It can be defined either as a relative value (%) or an absolute value (/kWh):

Similar to the total local consumer benefit, the total microsource benefit is composed of the locality benefit and the selectivity benefit. Figure 7.14 shows the maximum total benefit on the dispatchable microsource side in both absolute (/MWh) and relative (%) terms. Variations in microsource costs from 2010 to 2040 basically have negligible impacts on total benefit levels of all examined countries – this can be explained by looking at the maximum total microsource benefit simply as the difference between average retail and wholesale prices in an examined country, which stays largely constant despite microsource cost variation and market price changes. This will be discussed further in the sensitivity analysis of Section 7.4.
Figure 7.14 Maximum total microsource benefit, STC
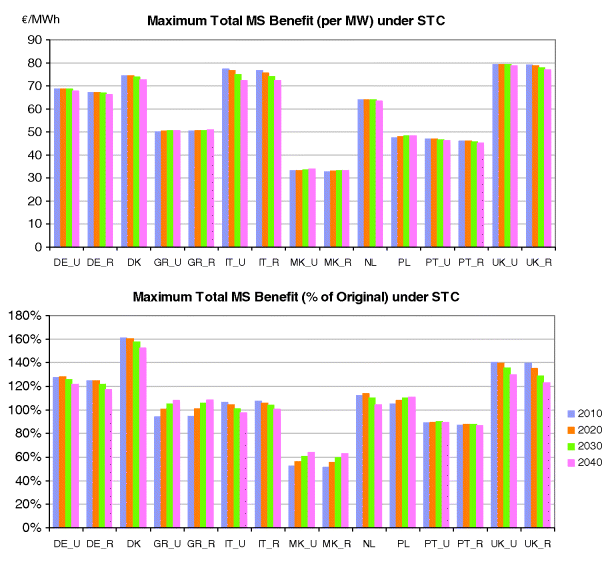
Microsource locality benefit is the difference between microsource cost and wholesale price level. It is actually an indication of “opportune operation loss” if the microsource units are forced to sell to the wholesale market instead of local consumers. None of the tested microgrid scenarios under a mid-level price and a real-time pricing setting could provide a direct microsource profit by selling directly to wholesale market.
The selectivity value on the microsource side equals the benefit obtained by consumer-side selectivity, that is incurred by energy consumption from local sources. To reach the full extent of selectivity benefit, consumers are expected to pay the same tariffs as before the microgrid implementation, and the DSO is not expected to charge locally consumed microsource energy for the grid usage. The selectivity benefit approximately yields the maximum achievable profit margin for the units. There is a very close correlation between microsource profitability and retail market price level, enabling high profits at 60–70 /MWh for high-price countries (e.g. Italy and UK) and much lower results around 20
/MWh for low-price countries (e.g. Greece and FYROM).
7.3.5 Environmental Benefits
The total emission reduction (regulator perspective) index is the reduction of GHG emission level per kWh consumption in a microgrid when compared to the passive grid operation. It can be defined either as relative value (%) or absolute value (/kWh):
Although SO2, NOX and particle matter emission reductions are also expected from microgrid operation, there are currently no explicit trading platforms within Europe as the European ETS market, thus reduction effects under these criteria can be viewed largely as by-products of GHG emission control.
Figure 7.15 demonstrates the environmental benefits of microgrids in absolute and relative values. Microgrid GHG emission levels generally converge to around 200 kg (CO2 equivalent)/MWh by 2040, despite very different starting points in 2010 – this convergence can be explained by the high resemblance of load-side self-sufficiency level in 2040. GHG reduction credits of microgrid are represented as a percentage of the original emission levels of each examined country. It is quite obvious that countries starting with high emission levels could expect reduction credits as high as over 50% (e.g. Greece and Poland), while countries with lower initial emission (e.g. Italy) enjoy comparatively smaller credits by 2040.
Figure 7.15 Average microgrid GHG emission level and emission saving credit, STC
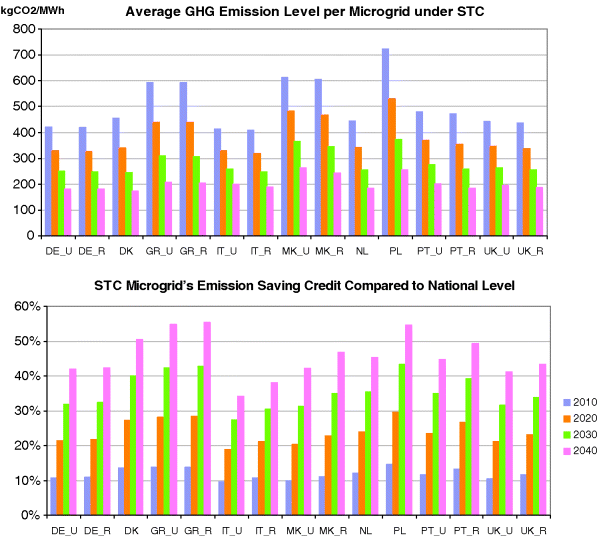
7.3.6 Reliability Improvement
With sufficient local generation and storage capacity, a microgrid can operate in islanded (off-grid) mode serving either the entire load or part of the load, namely the critical loads. The technical requirements of the power electronic interfaces of the DER to enable effective transition from interconnected to islanded operation and to sustain operation of a microgrid in islanded mode were discussed in Chapter 3.
An optimum level of reliability is defined mainly during the planning phase of microgrids, when costs for additional investments and operation are compared to avoided outage costs. Depending on the interruption costs and the given reliability of supply for networks without any microsources, different microgrid reliability benefits can be achieved at European level.
7.3.6.1 Economic Benefits Achieved by Reliability Improvement
Figure 7.16 compares the maximum economic benefits for different networks obtained by improved reliability. The x-axis represents the product of the total load of the network times the unavailability of this network in each year, symbolized by PQ. Benefits in each country are almost linear in relation to PQ, as interruption costs without DG increase with increasing total demand and unavailability. This leads to higher benefits of microgrid operation. The higher the outage costs, the higher economic benefits that can be achieved, as shown by the maximum, average and minimum interruption cost model.
Figure 7.16 Economic benefit comparison of microgrids on European level
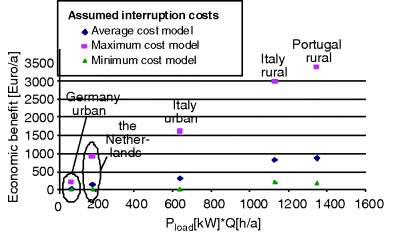
7.3.6.2 System Reliability Indices
A reduction of system unavailability Q, by the installation of microgrids that enable (partial) islanded operation, is demonstrated in Figure 7.17 for selected European networks, compared to the case without microsources.
Figure 7.17 System unavailability comparison of different countries
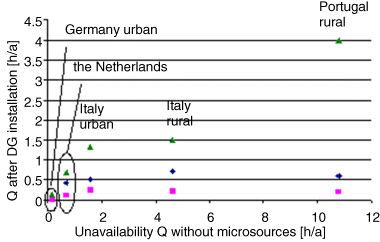
As expected, networks with lower reliability achieve higher improvements by the operation of microgrids, than networks of higher reliability. For instance, in the Portuguese rural network the system unavailability decreases from more than 10 h/a to below 1 h/a with the maximum and average cost model; with the minimum cost model, however, the annual unavailability is reduced to approximately 4 h/a. The improvement in the German urban network and the Dutch network, which have good reliability without microsources, are not significant, although the system reliability is also improved to a certain extent in both networks.
Thus, from the reliability point of view, microgrid operation is most beneficial in networks with lower power quality or for customer segments with comparatively high outage costs.
7.3.6.3 Optimum DG Penetration Level
The optimum DG penetration level is defined as the share of the local DG units required to achieve a minimum number of supply interruptions. As expected, the higher the DG penetration level, the lower the interruption frequency. Specifically, the function of the optimal DG penetration level from reliability perspective is almost linear to DG installed capacity (Figure 7.18). This relationship is important for system planning; that is, as the system interruption frequency without DG istallation is generally known, the DSO is able to decide the optimum DG penetration level from a reliability point of view.
Figure 7.18 Optimal DG penetration for minimum interruption frequency considering average interruption costs
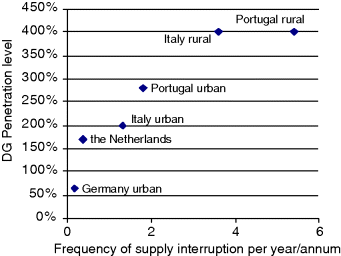
7.3.6.4 Optimum Microsource Location from Reliability Perspective
Investigations show that when only failures caused at the LV network are considered, the optimal microsource location should take into account the following criteria:
- distributed in different protection zones
- located most downstream in the network
- connected close to the load with higher demand
- prioritized to supply sensitive loads
When failures at the MV and HV level are also considered, different microsource locations have the same effect on the frequency of load interruption; in this case, microsources improve system reliability regardless of their location in the LV grid.
7.3.7 Social Aspects of Microgrid Deployment
Three major social benefits of microgrids can be identified as follows:
7.3.7.1 Raise Public Awareness and Foster Incentives for Energy Saving and Emission Cutting
Depending on the microgrid model, DER-created values are shared among end consumers of a network. Residential and commercial participants of a microgrid will likely enjoy better electricity tariffs and can even be rewarded by incentive programs if they own RES microsources or participate in demand-side integration (DSI) programmes. This type of economic signal (efficiency values created within a microgrid) can be seen as a strong driving force for acceptance and promotion of microgrids.
7.3.7.2 Creation of New Research and Job Opportunities
Microgrid implementation requires knowledge, expertise and customized hardware and software solutions that are currently not directly available for both supply- and demand-side players in the market. Opportunities for new research posts and job openings will be offered for technology providers, device manufactures, research institutes, and so on. Opportunities will become available not only in the design (stereotyping) and installation (standardization) stages, but also in daily operation and maintenance of a microgrid, for example requirements for real-time metering, communication and control both within and outside of microgrids.
7.3.7.3 Electrification of Remote or Underdeveloped Areas
For a long time, it has been widely acknowledged that DER units are extremely well suited for electrifying remote or underdeveloped regions, where it is not economic to interconnect to a nearby distribution grid or where there is simply lack of basic electric infrastructure. The microgrid concept provides a platform for aggregating isolated sectors of self-sufficient households or communities based on limited micro-generators and storage units into a more robust network with balancing and control capacities. Reliable and affordable supply of electricity via microgrid can be seen as a critical step for modernization and industrialization of the local economy.
7.4 Impact of External Market Prices and Pricing Policies
7.4.1 Sensitivity Analysis
7.4.1.1 External Market Price Policies
External market prices have a critical impact on microgrid profitability. In Figure 7.19, three potential price settings are assumed, as inputs to the sensitivity analysis of microgrid profitability (see also Section 1.5.3 and Table 1.1):
- Pricing policy 1 (fixed prices): directional + constant pricing: economic island model
- Pricing policy 2 (flexible prices): directional + variable (ToU) pricing: hybrid model
- Pricing policy 3 (uniform prices): uniform + variable (ToU) pricing: exchange model.
Figure 7.19 External price policies applied to microgrids
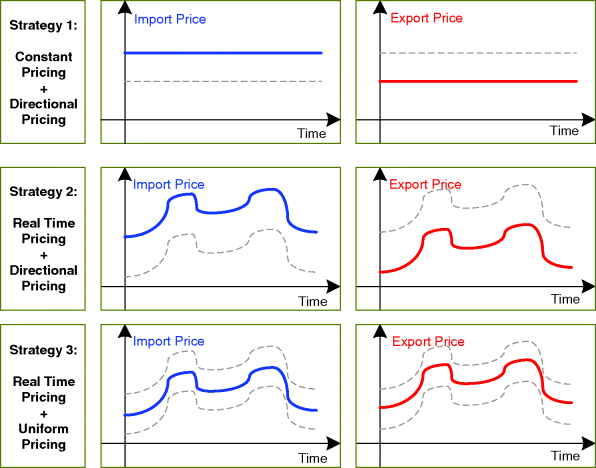
7.4.1.2 Wholesale Market Price Levels
Due to the extremely volatile nature of wholesale electricity prices, it is not clear whether future electricity prices will increase (e.g. due to scarcity of conventional resources) or decrease (decreasing demand due to higher efficiency) compared to their current level. Therefore, electricity price levels (annual average of wholesale price in all countries) are assumed to be constant, irrespective of time, and three general price levels are assumed for all countries and for all scenarios considered. Figure 7.20 shows the annual average values of high, mid and low prices at wholesale level, respectively, as annual average values for the analyzed countries. The high price scenarios are taken as equal to the peak prices from the past decade, while mid and low cases are respectively calculated as 80% and 60% of peak value.
Figure 7.20 Three wholesale price levels assumed for microgrid evaluation
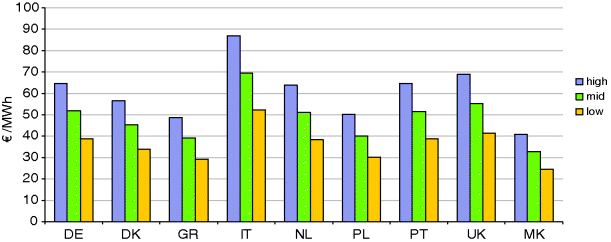
In order to ensure microgrid profitability under low market prices, market acceptance of local consumption satisfaction is assumed here for all cases.
7.4.2 Sensitivity of Energy Balancing in Response to External Market Prices and Price Settings
Figure 7.21 shows the effects of market price levels on consumer-side self-supply level. It is obvious that low external market price levels invariably lead to reduction of microsource production and reduction of microgrid self-sufficiency, while the opposite holds – for high market prices. Cases with low to mid prices (such as Greece, FYROM, Poland and Portugal) are much more sensitive to annual wholesale price changes (up to ±15%) compared to higher-price cases (largely below ±5%).
Figure 7.21 Market price and pricing policy impact on consumer-side self-supply
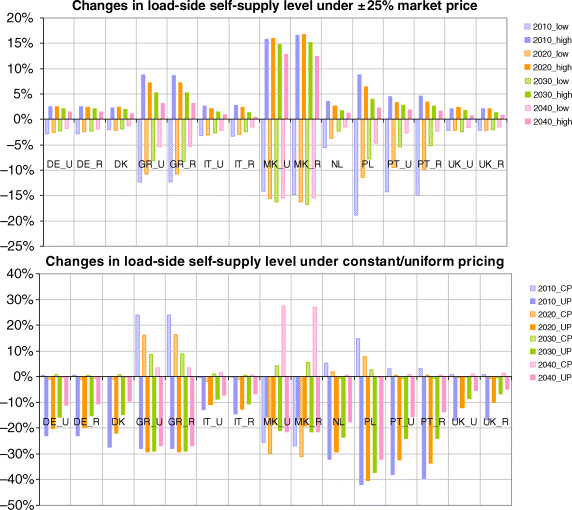
Compared to real-time pricing, application of fixed prices has a negligible impact on higher-price cases, but it visibly increases self-sufficiency level of low- to mid-price cases. Recognition of locational value (uniform pricing), however, always leads to lower self-sufficiency levels due to a general reduction of retail purchase tariffs.
Figure 7.22 shows the effects of external market price changes on the microsource side of self-supply level.
Figure 7.22 Market price and pricing policy impact on microsource contribution to self-supply
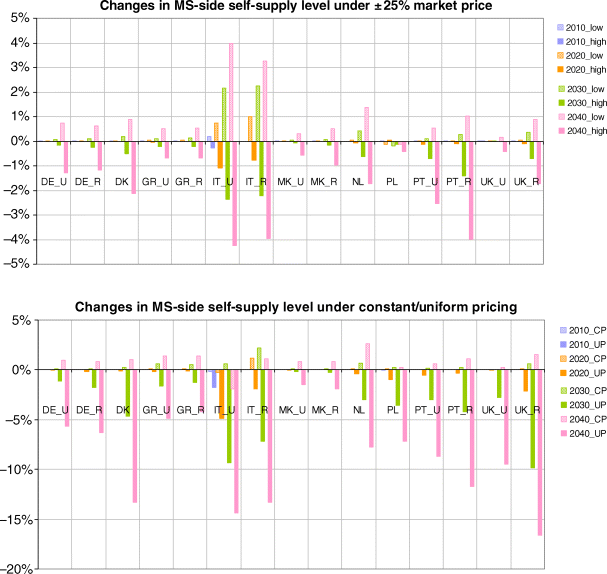
In contrast to the consumer-side self-supply level, the microsource contribution to self-supply increases with lower market price levels and decreases as average market prices increase. This can be easily explained as prices determine export opportunities during peak price hours. In a number of cases, microsource contribution to self-supply level varies by ±4%, while in most of them this falls within ±1%, which indicates a very small impact of market prices on microsource export opportunities. This suggests that export opportunities, even with a high penetration of local DER (2040 case) will be primarily determined by the load level instead of the external price level. Fixed pricing has similar effects as it leads to market prices variations generally within ±2%. Uniform pricing, however, results in considerably larger reduction of microsource self-supply, thus increasing exports to the upstream network by 10–15% in 2030 and 2040 scenarios.
The effect of market price levels and pricing policies on microsource dispatch, expressed as mean FLH, can be seen in Figure 7.23. The impact of market price level on microsource FLH shows a very similar trend to that of the consumer-side self-supply index shown in Figure 7.21. FLH of low-price cases exhibits higher sensitivity to average wholesale price variations (up to ±60% in the case of FYROM), while high-price cases (e.g. Germany, Denmark, Italy, the Netherland and the UK) generally experience variations less than ±10% and are hardly impacted by wholesale market price levels.
Figure 7.23 Market price and pricing policy impact on microsource full load hours
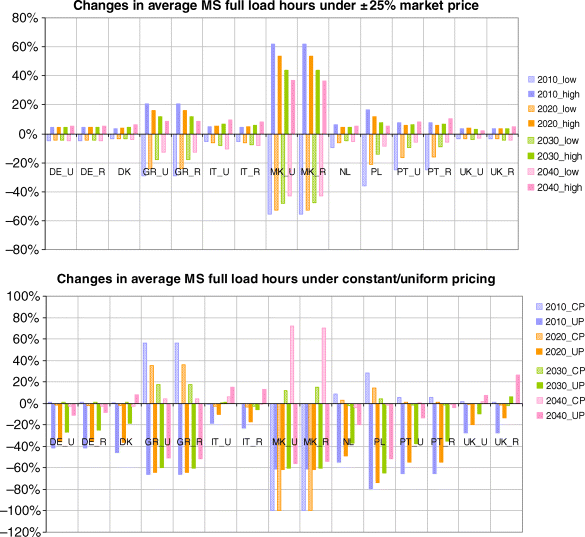
Fixed pricing, as defined in Section 1.5.3, has negligible effects on high-price cases, but can increase DER FLH by as much as 80% compared to real time (ToU) pricing for low-price cases, such as FYROM, Greece and Poland. This can be understood as the benefit of price stability in adverse market settings. Uniform pricing provides a 20% to 80% FLH reduction in 2010, which is decreased drastically with time; for example, in some cases FLH starts to increase by 2040 as a result of uniform pricing.
The effects of market price levels and pricing policies on the required premium support level for RES can be seen in Figure 7.24. The support levels are calculated as the difference between mean RES production cost and average retail prices. Owing to the linear correlation between market prices and required RES support, variations in average wholesale prices level induces proportional changes in required RES support, that is higher prices lead to reduction of required support and vice versa. In general, a ±25% market price variations leads to a change in required RES support by 10–20%.
Figure 7.24 Market price and pricing policy impact on RES premium support level
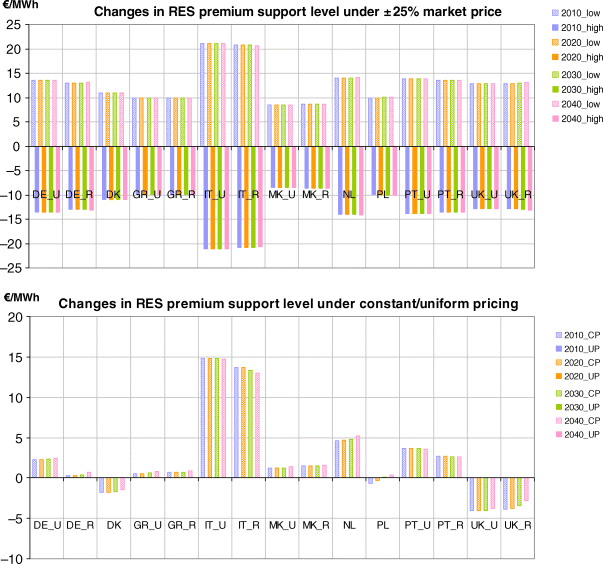
Fixed (constant) pricing leads to an increase in the required premium support for some countries (e.g. DE, IT, NL and PT) and lower support for others (e.g. DK, PL and the UK). Uniform pricing, on the other hand, is assumed not to interfere with RES support and consequently has no impact on required national support levels.
7.4.3 Sensitivity of Technical Benefits (Losses) in Response to External Market Prices and Price Settings
Figure 7.25 shows the impacts of market price levels and price settings on losses. The trends are similar to those of the load-side self-sufficiency level index of Figure 7.21, since losses are roughly dependent on the levels of microsource exports for all examined simulation cases.
Figure 7.25 Market price and pricing policy impact on loss reduction credit
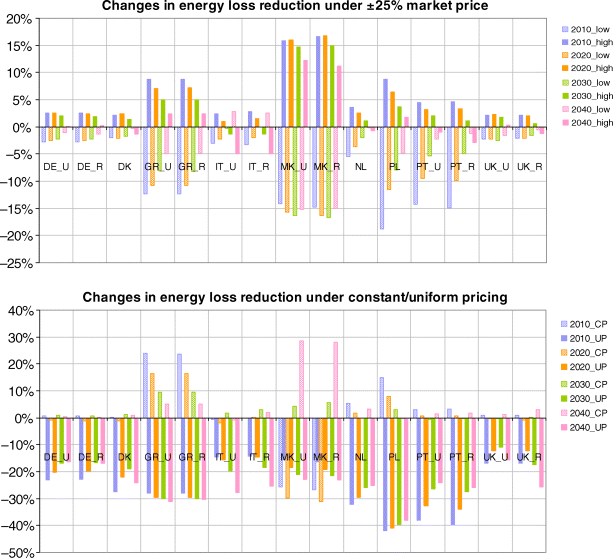
7.4.4 Sensitivity of Economic Benefits in Response to External Market Prices and Price Settings
7.4.4.1 Consumer Side
The effects of market price variations and different pricing policies on the total economic benefit on the consumer side are shown in Figure 7.26. Differences between the various cases under the same wholesale price variations are comparatively small, indicating a benefit variation ±20% to ±40% for a ±25% variation of market prices. Fixed pricing has mostly negative impacts on maximum consumer benefit, with small benefit reductions under 10% for most cases except FYROM, where a benefit reduction as high as 35% is calculated due to a drastic reduction of microsource full load hours (even to zero). Uniform pricing could significantly raise the maximum total consumer benefit by 2–6 times initially in 2010, but this increase is quickly damped with time.
Figure 7.26 Market price and pricing policy impact on maximum load-side benefit

7.4.4.2 Microsource Side
The potential effects of market price variations and pricing policies on the maximum total benefit of microsources are shown in Figure 7.27. The total microsource benefit is almost solely determined by the difference between the selling price and the average wholesale price in the market. Wholesale market price levels and fixed pricing schemes have basically negligible impacts (below 5%) on its value except the case of FYROM. Uniform pricing, however, is potentially capable of changing the mean microsource selling price and can therefore lead to total microsource benefit changes as high as ±50% to ±100%.
Figure 7.27 Market price and pricing policy impact on maximum microsource side benefit
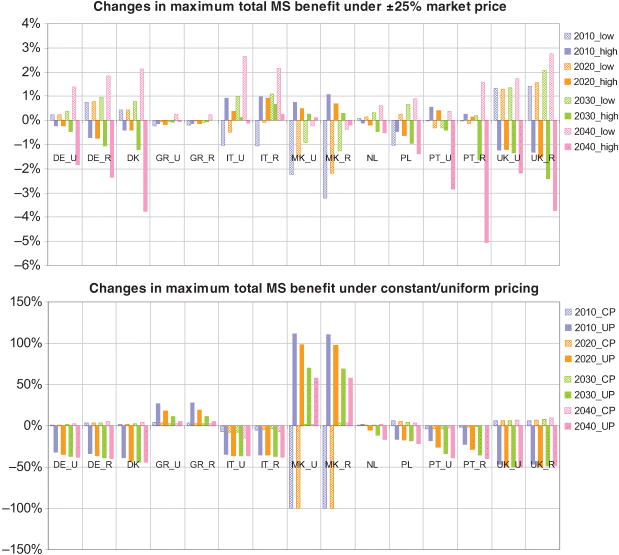
7.4.5 Sensitivity of Environmental Benefits in Response to Market Prices and Price Settings
The effects of market price levels and pricing policies on microgrid emission savings are shown in Figure 7.28. There is a close correlation between microgrids self-sufficiency level and its emission reduction credit. There are different national emission level baselines and large variations of emission levels in response to external prices and pricing settings, but the actual impact of both criteria is comparatively small (less than ±10%). The only exception is the effect of uniform pricing, which could potentially lead to a reduction of more than 25% in terms of GHG savings compared to real-time pricing.
Figure 7.28 Market price and pricing policy impact on emission savings
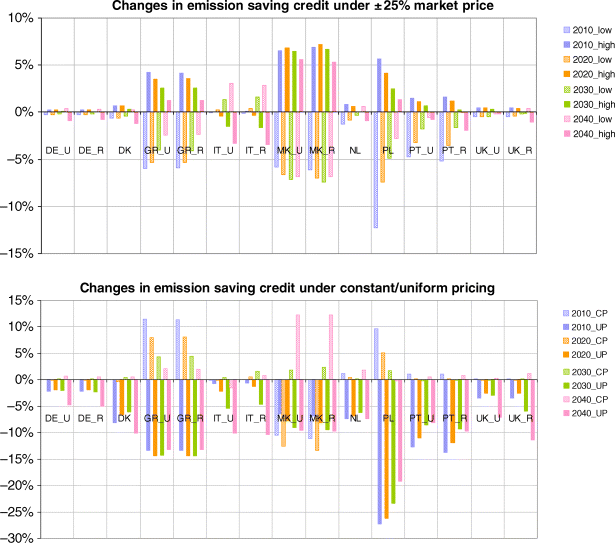
7.5 Impact of Microgrid Operation Strategy
Figure 7.29 compares the maximum total consumer benefit obtained from the combined operation mode (the objective function is the summarized total cost, and all technical constraints are applied) used as a reference to the following three individual operating strategies for one sample microgrid (UK urban) in 2010 to 2040 scenarios:
- Economic strategy: objective function is the maximization of profits as seen from microsource aggregator perspective; no network technical constraints are applied.
- Technical strategy: objective function is the minimization of losses; all technical network constraints are applied as seen from a DSO perspective.
- Environmental strategy: objective function is the minimization of emissions from a regulator perspective; no network technical constraints are applied.
Figure 7.29 Sample microgrid operation mode impact over different self-supply levels
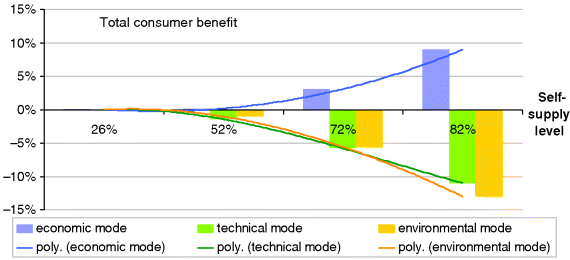
It can be seen that the differences between the strategies are not significant when the self-supply level of a microgrid is below 50%.
As expected, multi-objective optimization provides a balanced set of benefits, while the single-objective optimizations provide higher benefits for the respective individual benefit at the expense of the other benefits.
In order to facilitate illustration, the following primary indices are defined:
These indices are calculated for each of the three strategies and compared to the corresponding indices obtained from the combined multi-objective optimization for the studied European microgrids. The relative differences are plotted respectively in Figures 7.30–7.32 as percentage values where the multi-objective calculation results are taken as reference (i.e. the denominator).
Figure 7.30 Operation strategy impact on maximum economic benefits
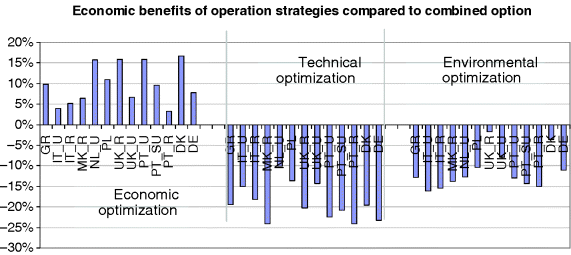
Figure 7.31 Operation strategy impact on energy losses
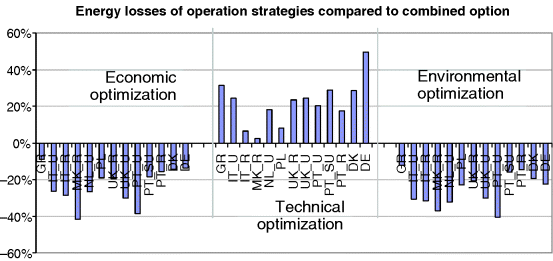
Figure 7.32 Operation strategy impact on GHG reduction credits
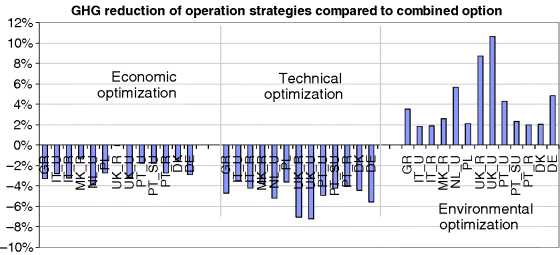
It can be clearly seen that the combined multi-objective optimization provides a balanced satisfaction of all objectives and can be physically viewed as a compromise between the different microgrid stakeholders in order to arrive at the global optimum of economic, technical and environmental performances.
In Figures 7.33 and Figure 7.34, the voltage and loading reduction indices are compared for all examined cases. It can be seen that the combined strategy achieves the same level of network performance as the technical optimization strategy, while the economic and environmental optimization strategies fail to do so.
Figure 7.33 Operation strategy impact on loading reduction
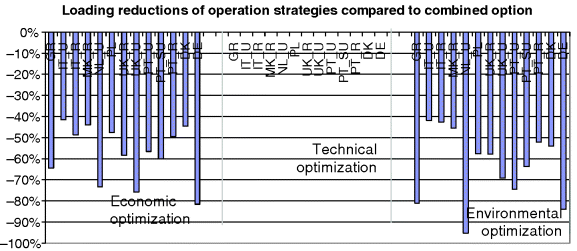
Figure 7.34 Operation strategy impact on voltage variation
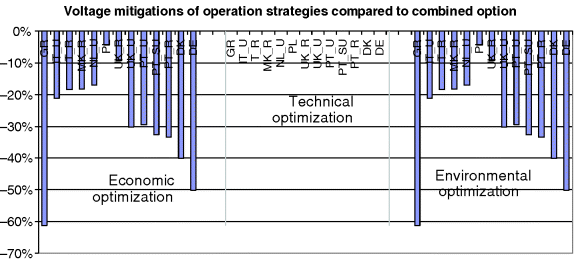
7.6 Extension to European Scale
As shown in Section 7.2 the microgrid self-supply level is the most important factor affecting the benefits that it provides to the various stakeholders. Figure 7.35 demonstrates the maximum total consumer benefit for the various European study case networks as a function of self-supply level. Benefit increases as the microgrid self-supply level increases and reaches 35 ±25 /MWh at 90% (consumer-side) self-supply level. This maximum benefit is the sum of potential price reductions due to local trading, as well as network and emission charge reductions. The values shown are based on the assumption that all economic benefits are obtained by the end consumer. In practice, it is expected that microsources, DSO and suppliers or other potential intermediary parties (e.g. ESCOs) will very likely share this total benefit.
Figure 7.35 Summarized total consumer benefit as a function of load self-supply
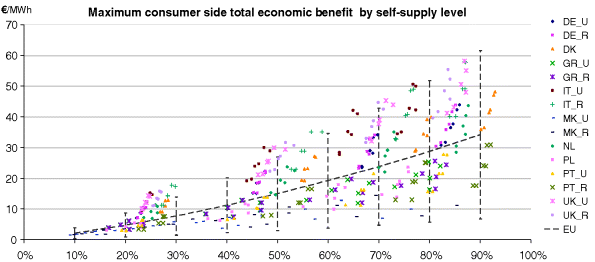
Figure 7.36 summarizes the maximum consumer benefit due to retail market selectivity for the various European study case networks. These benefits reach 30 ±20 /MWh when dispatchable microsource units can supply 50% of microgrid demand (i.e. the same time as 90% total self-supply level, when the remaining 40% load is supplied via intermittent RES units). Comparison with Figure 7.35 indicates that economic values created from market selectivity are expected to provide higher shares of total benefit as self-supply level rises.
Figure 7.36 Summarized consumer benefit due to microgrid selectivity in a European context

Figure 7.37 demonstrates that the maximum total microsource benefit can reach about 60 ±30 /MWh for the various European study case networks. This level of benefit is largely independent of microgrid self-supply level, as the total economic benefit of microsources is solely determined by the difference between wholesale and retail prices applicable to the microgrid environment. Similar to the consumer-side benefits, the actual benefits received by the microsource owners are likely to be smaller, since they can be potentially shared with other involved parties.
Figure 7.37 Total microsource benefit as a function of microgrid self-supply
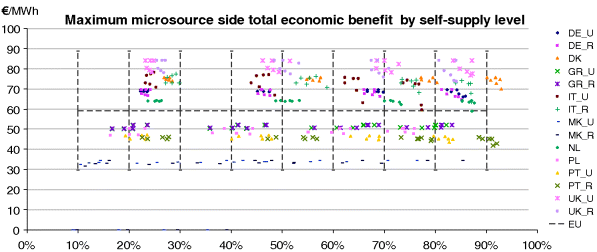
Figure 7.38 summarizes the ideal loss reductions for the various European study case networks. A reduction of about 75% ±20% can be expected at 90% self-supply level.
Figure 7.38 Summarized loss reductions
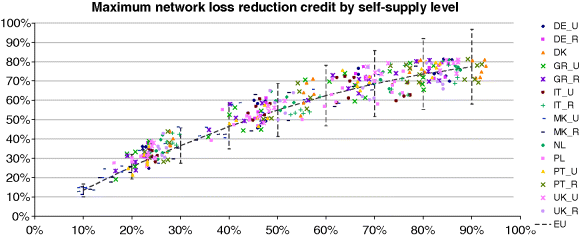
Figure 7.39 summarizes the ideal voltage regulation improvement for the various European study case networks. A reduction of 50% ±15% can be expected at 90% self-supply level.
Figure 7.39 Voltage regulation
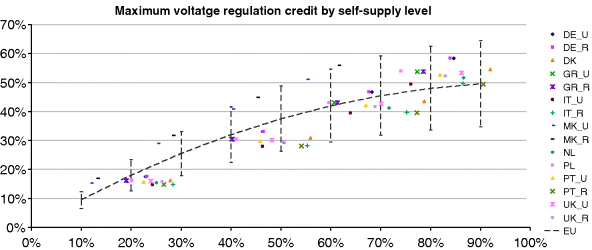
Moreover, a reduction of about 40% ±12% of the peak load at 90% self-supply level (Figure 7.40) and a reduction of GHG emissions by about 55% ±25% at 90% self-supply level (Figure 7.41) can be achieved under an ideal setting.
Figure 7.40 Peak load reduction
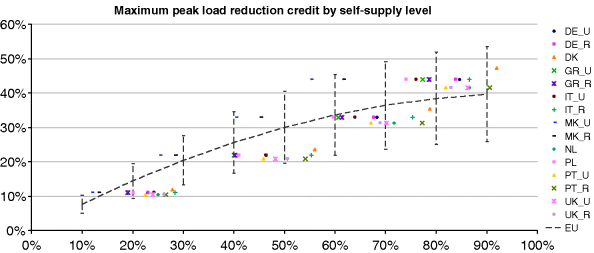
Figure 7.41 GHG emission reduction

7.7 Conclusions
Network operation under a microgrid paradigm provides novel possibilities for overcoming the conflicting interests of different stakeholders and for achieving a global socio-economic optimum in the operation of distributed energy sources. Economic, technical and environmental impacts of microgrids are closely related to the operating decisions about the outcomes of microsources, storage units and DSI programmes. Proper planning of a microgrid requires knowledge and simulation of its potential operating conditions, while at the same time different planning decisions; especially those related to DG/RES penetration level, will critically affect potential benefits of a microgrid.
In this chapter, microgrid benefits have been identified for a number of representative distribution grids using a variety of input data from representative European countries and regions. In order to quantify the benefits obtained from different real-time operating conditions, each case study network has been simulated as a consecutive day-to-day scheduling problem with annual sets of stochastic weather, market and demand data. A multi-objective optimization algorithm has been used that incorporates technical and environmental aspects either as economic objectives or as operational constraints. An optimal power flow technique is used in combination with meta-heuristic methods to estimate real-time system states. Finally, reliability improvements and social benefits have been considered independently from daily microgrid operation and are thus studied separately.
Study results indicate that broad ranges exist for literally all economic, technical and environmental benefits potentially obtained from microgrids in different countries and regions. Considering the high uncertainties in the underlying assumptions, it is very difficult to provide reliable, quantified benefits of satisfactory precision. Despite individual differences, however, general trends of microgrid effects can be safely observed. Statistical analysis of the calculated results also suggests that the energy self-sufficiency level (demand-side) is a good indicator of the potential values of most benefit indices.
The main results of the benefits studies are as follows:
Benefits of Microgrid Operation
A microgrid could potentially offer economic benefits (single or multiple from list) such as:
- price reduction for end consumers,
- increased revenues for microsources,
- investment deferral for distribution system operators.
Economic benefits arise mainly from locality values created by local retail market and selectivity values created by optimized real-time dispatch decisions.
To achieve the expected economic benefits, it is necessary to recognize local (over-the-grid) energy trading within a microgrid, to apply real-time import and export prices for microgrids and to have an (optimal) RES support scheme and favorable tariffs.
A microgrid could potentially offer technical benefits (single or multiple from list) such as:
- energy loss reduction,
- lower voltage variation,
- peak loading (congestion) relief,
- reliability improvement.
Technical benefits can be either traded in a local service market between microsource and DSO or implemented as price signals. To achieve the expected technical benefits, optimal dimensioning and allocation of microsources as well as coordinated multi-unit microsource dispatch, based on real-time grid conditions is necessary.
Environmental benefits of microgrid can be mainly attributed to:
- shift toward renewable or low-emission fuels used by internal microsources,
- adoption of more energy-efficient technologies, such as CHP,
- adoption of DSI options.
Social benefits of microgrids can be summarized as:
- raising public awareness and fostering incentive for energy saving and GHG emission reduction,
- creation of new research and job opportunities,
- electrification of remote or underdeveloped areas.
1. More Microgrids (2006–2009) Advanced Architectures and Control Concepts for More Microgrids, FP6 STREP, Contract no.: PL019864., http://www.microgrids.eu.