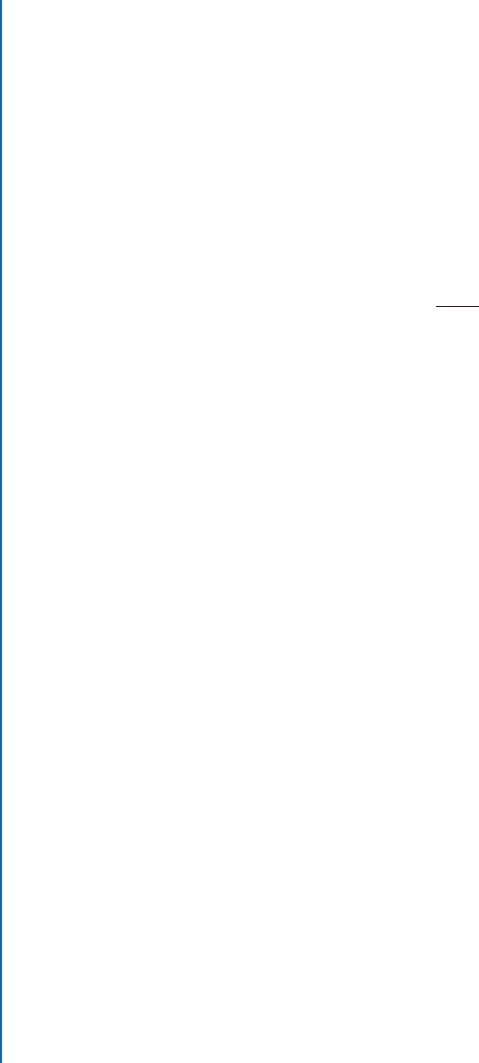
156 11. FIELDS
If the mass of a neutron star were to be compressed to only slightly smaller volume, it
would form a black hole—an object so dense that the extreme curvature of space prevents even a
light beam from escaping it.
If mass is confined within a certain radius such that even light cannot escape the gravity,
then a black hole is formed. is radius is known as the Schwarzschild radius, and it sets the
effective size of a black hole. And so we imagine a sphere with a radius equal to the Schwarzschild
radius. e surface of this sphere is called the event horizon—anything that passes through it
cannot return.
e Schwarzschild radius, R
S
, of a black hole depends only upon its mass, M , and it has
a surprisingly simple formula:
R
S
D
2GM
c
2
; (11.13)
where G is the gravitational constant and c is the speed of light. If we express M in units of the
mass of the Sun, the Schwarzschild radius is approximately 3 km for every solar mass.
Black holes greatly alter the spacetime nearby, but at large distances their gravity is not
significantly different from what it would be if the same amount of mass were not compact and
in the form of a black hole.
e black holes that remain from the collapse of massive stars are very difficult to detect; it
is practically impossible for a solitary black hole. But in a close binary star system, the companion
star may transfer matter to the black hole, and this produces X-rays that are detectable. ere
are several such examples known.
But by far the most easily detectable black holes are the supermassive black holes found
at the centers of many large galaxies. Figure 11.6 shows the first successful “photograph” of a
supermassive black hole—in the center of the giant elliptical galaxy M 87.
is remarkable image was released in April, 2019 by the Event Horizon Telescope team.
e dark hole in the center of the image is larger than the Schwarzschild radius of the black hole.
It represents the edge of the ergosphere—a region around a black hole where light beams would
essentially orbit the concentration of mass. e mass calculated for this supermassive black hole
is about 6.5 billion times the mass of the Sun.
11.2.3 DARK MATTER AND DARK ENERGY
e nature of dark matter (see Section 5.4.5) is consistent with a type of matter that behaves, so
far as gravity is concerned, the same as any other matter. It is its interactions with other known
forces (electricity, magnetism, and the forces within the nucleus of an atom) that are unusual.
But so-called dark energy (see Section 5.4.6) is another kettle of fish. Dark energy is the name
for the recent observation that the universe is accelerating its expansion, rather than slowing
down as one would expect from “normal” gravity. e cause of this acceleration is unknown, but
it has a deep connection, historically if nothing else, to Einstein’s GR.